Photothermal effect and application of photothermal materials in photocatalysis and photoelectric catalysis
Abstract
Photocatalysis (PC) and photoelectric catalysis (PEC) are environmental protection technologies that use sunlight capacity and environmental governance, and they have a wide range of applications in hydrogen production, carbon dioxide reduction, organic degradation, and other fields. When the light is irradiated on the material, part of the light energy will be converted into heat energy, and the combination of this part of the heat energy with PC and PEC will become an important way to improve optical performance. Compared with traditional technology, the synergistic effect of light and heat can obtain higher catalytic performance and improve energy utilization efficiency. This review begins with an overview of the principle of photoheat generation, which produces heat energy in a non-radiative process through photo-induced instability of electrons. The principle of thermal effect on the performance improvement of PC/PEC is analyzed from the dynamics and thermodynamics of photoreaction and electric reaction. On this basis, several materials widely used at present are listed, such as oxides, plasmas, conductive polymers, carbon materials, and other typical photothermal materials. The specific applications of photothermal materials in PC and PEC processes, such as hydrogen production by oxidation, carbon dioxide reduction, organic matter reduction, and seawater desalination, were discussed. Finally, the challenges to PC/PEC from the introduction of thermal effects are further discussed to provide a clean and sustainable way to build a carbon-neutral society.
Keywords
INTRODUCTION
With the development of the world economy and the increase of population density, the world is facing two major crises: one is energy demand, and the other is environmental pollution[1-3]. To develop sustainable green energy instead of traditional fossil energy becomes an effective way to solve the energy crisis[4-6]. At the same time, reducing carbon dioxide emissions through efficient and energy-saving ways can also effectively mitigate environmental pollution[7,8]. Solar energy is the most abundant renewable energy on earth. The utilization of solar energy by photosynthesis in nature provides a solution for us. A shift from fossil fuels to clean energy, such as hydrogen, could significantly reduce carbon dioxide emissions[9,10]. In 1972, Fujishima and Honda first proposed to use TiO2 as photoanodes for water splitting[11]. Using the energy level transition of semiconductors under sunlight to decompose aquatic hydrogen or reduce carbon dioxide has become an efficient means of solar energy conversion. Common semiconductor materials include TiO2[12], WO2[13], BiVO4[14], Fe2O3[15], Cu2O[16], ZnO[17], etc. All of them have different performance characteristics in photocatalysis (PC)/photoelectric catalysis (PEC).
The process of photocatalytic reactions is to excite electrons from the valence band (VB) to the conduction band (CB) by using the excitation of light on the semiconductor material to form an electron-hole pair[18]. On this basis, PC promotes the separation of electrons and holes through applied voltage[19]. The activity of photogenerated charge carriers is largely determined by the thermodynamics and kinetics of semiconductor materials[20]. In thermodynamics, the photoexcitation makes the chemical potential of the electron system higher than that of the hole system, making ΔG negative, thus generating the photovoltage[21-23]. In terms of dynamics, defects and doped atoms in semiconductor materials can act as charge transfer carriers to accelerate electron transport and may also act as charge aggregation centers to affect the recombination rate of electron holes[24-26]. The current modification techniques mainly focus on the structure of the material itself, including band gap doping[27], building nanostructures[28], loading cocatalysts[29], building composite heterostructures[30], and other strategies. The principle of its modification is to accelerate the reaction kinetics and reduce the thermodynamic electromotive force, thus inhibiting the surface recombination of electrons and holes, forming a higher photogenerated current, accelerating the catalytic reaction process, and improving the stability of the catalyst[31,32].
However, the improvement of the material performance through structural modification alone has its limitations. Therefore, the researchers use other systems to enhance the catalytic performance[33]. Several common systems reported today include dual electrodes[34], photovoltaic photoelectrochemical (PV-PEC) systems[35], and semiconductor-based physical effects such as photothermal[36], pyroelectric[37], ferroelectric[38], and piezoelectric[39] effects. Using the physical effects of the material itself is a more refined approach than other electrochemical water decomposition systems. In catalytic reactions, reducing and utilizing the heat loss of the reaction can better improve the utilization rate of the reaction, so the photothermal effect has become an important direction of research[40-42].
Photothermal effects mean that after the material is irradiated by light, the photon energy interacts with the lattice, making the vibration intensified, thus increasing temperature[43]. Under the photothermal effect, the semiconductor absorbs and concentrates heat from the photons, and the thermal energy generated by the photoexcitation can heat the local lattice and enhance the vibration of the phonons. Theoretically, raising the temperature will increase the collision probability of the active molecules, promoting the dynamic process of the thermodynamic uphill reaction[44-46].
This paper reviews the application of photothermal effects in PC/PEC systems. Firstly, the principle of photothermal effects improving photocatalytic performance is discussed, along with an examination of various photothermal materials. Then, the paper separately explores the applications of photothermal materials in photocatalytic and photocatalytic systems. Figure 1 shows the review process of the research progress of photothermal materials in this paper.
PRINCIPLE OF PHOTOTHERMAL EFFECT
The thermodynamics of PEC processes determine whether the reaction can proceed. The process of hydrogen production by PEC cracking water is to carry out redox reactions between the photogenic carrier generated by solar radiation on the catalyst surface or the active site of the catalyst and the solution to achieve hydrogen and oxygen production [Figure 2A][47,48]. In the research system of photoreaction, ultraviolet (UV) light is the main band of sunlight that acts on the photoexcitation of semiconductors. However, UV light accounts for only about 5% of the solar spectrum, and extending light absorption to the longer wavelength visible or even infrared light region can effectively use the energy of the spectrum. The visible light to infrared light region brings stronger photothermal effects in the process of PC[49,50]. High utilization rates of light and good photothermal effects have become the consensus of improving energy conversion efficiency, but there are many different theories on the mechanism of photothermal effects on photocatalytic performance[51].
Figure 2. (A) The illustration of PEC water splitting using a photoanode[53]. Copyright 2022, Elsevier Ltd. (B) The main recombination paths of semiconductor photogenerated holes and electrons[57]. Copyright 2013, Royal Society of Chemistry. (C) TAS decay traces of the photohole in α-Fe2O3 photoanodes at different temperatures fitted to single-exponential functions. (D) Arrhenius plots of the hole decay on α-Fe2O3 photoanodes under various applied biases[63]. Copyright 2011, American Chemical Society.
As shown in Figure 2B, the principle of photothermal effects can be analyzed from the molecular point of view. Photothermal materials make electrons enter the excited state by absorbing photons. In the excited state, electrons are unstable and undergo a series of deexcitation processes. In these processes, part of the non-radiative process will be converted into heat energy and emitted, that is, ultimately manifested as the process of converting light energy into heat energy[52-54].
Effect of temperature on equilibrium and rate of chemical reaction
Water splitting as the content of photocatalytic reactions is analyzed. One molecule of H2O can be converted to H2 and 1/2 O2 in the following reaction:
Therefore, PC is a typical energy uphill reaction, which needs to absorb heat. Based on the analysis of the basic physicochemical mechanism, the temperature adjustment can facilitate the equilibrium movement of chemical reactions, thus regulating the water decomposition performance of PEC[55-57].
In 1884, Van't Hoff proposed the relationship between the equilibrium constant K of a chemical reaction and the change of temperature T in the Van't Hoff equation[58]:
(K is the equilibrium constant, ΔH is the enthalpy change, R is the gas constant, and T is the temperature).
It can be seen from the equation that for endothermic reactions, with the increase of temperature, PEC reactions will move in the positive direction, thus producing more hydrogen and oxygen.
In several reaction steps of PC processes, the oxygen evolution reaction (OER) is the slowest and rate-determining step (RDS) in the water splitting reaction[59,60]. From the relationship between the most basic chemical reaction rate and temperature, it can be inferred that temperature improves the catalytic reaction process. The Arrhenius formula shows the relationship between the chemical reaction rate constant and temperature[61]:
(k is the rate constant, R is the molar gas constant, T is the thermodynamic temperature, Ea is the apparent activation energy, and A is the pre-factor).
It can be seen from the equation that the pre-factor A determines whether the molecules collide in the right direction and only decides whether the reaction can proceed. The index
Cowan et al. used transient absorption spectroscopy (TAS) to investigate the temperature-dependent decay of photoluminescence holes in α-Fe2O3 during PEC[63]. As shown in Figure 2C, the photogenerated hole decay rate of α-Fe2O3 increases with rising temperature. The decay rate of photoluminescence holes is positively correlated with temperature under different bias pressures [Figure 2D].
Influence of thermal effects on electrochemical processes
A PEC process is characterized by light-electrochemistry interactions under an applied light that generates electron excitation following charge transfer from photoexcited materials. Therefore, it can be used as an electrochemical reaction, and its reaction process can be analyzed by an electrochemical reaction equation[64]. The Nernst equation is used to describe the quantitative relationship between the electromotive force (E) electrolyte concentration of a battery. For the electrochemical process of water decomposition, the Nernst's equation can be written as[65]:
From ΔG0 = 237.2 kJ mol-1 of the water splitting reaction, the corresponding PEC initial potential (Ecell) should be -1.23 V according to the Nernst equation[55]. When the temperature of the reaction system increases, the initial potential of PEC decreases. Under the same light and bias conditions, it is more conducive to the movement of charge and promotes the catalytic reaction[66,67].
The paper of Ye analyzed the effect of temperature on the PEC performance of α - Fe2O3 photoanodes in water[68]. As shown in Figure 3A, the dark current density of α - Fe2O3 photoanode shifts at different temperatures. Figure 3B shows the temperature dependence of the starting potential of the electrode under light, and the starting potential is anodized with increasing temperature and decreasing doping level.
Figure 3. (A) The dark current density of hematite photoanodes with different temperatures. (B) Onset potential of a hematite photoanode as a function of the illuminated light intensity[68]. Copyright 2016, Stanford University (C) Temperature-dependent band gap energy diagram of a TiO2 photoelectrode[69]. Copyright 2023, Springer Nature.
Temperature effect of band gap width of semiconductor materials
At present, the widely used photocatalysts are essentially semiconductors. Semiconductor materials have a fixed band gap energy (Eg), and the band gap energy width is determined by the energy level of the VB and the CB, that is, the energy difference between the lowest point of the CB and the highest point of the VB[69]. In order to achieve water splitting to produce hydrogen and oxygen through PEC, the lowest potential of CB should be lower than the reduction potential of hydrogen (≤ 0.0 V vs. RHE), and the highest potential of VB should be higher than the oxidation potential of oxygen (≥ 1.23V vs. RHE)[70,71]. When a specific wavelength of light is irradiated on the catalyst, the absorption capacity of the PEC material is equal to or greater than the band gap energy of the semiconductor comprising the photoelectrode, which excites the electrons in VB to migrate to CB, correspondingly leaving a hole in VB to produce an electron-hole pair[67].
The Varshni's equation can describe the band gap change of a semiconductor with temperature, and its equation form is as follows[72]:
(Eg(T) is the band gap at temperature T, and α and β are the property constants of semiconductor materials).
According to the equation theory, the basic trend of material band gap with temperature change is as follows: as temperature increases, the band gap width of the material narrows[73]. Figure 3C shows the spectrum diagram of the TiO2 band gap width changing with temperature. As temperature increases, the band gap energy decreases[69].
Combined with literature analysis, the reasons for the band gap change of a semiconductor with temperature can be analyzed from two aspects: first, the increase of temperature and the expansion of the lattice inside the material. The second is that the temperature increases and the lattice vibration intensifies, resulting in a stronger interaction between the phonons and electrons in the semiconductor[74-76].
Temperature increases the carrier concentration in the semiconductor
The performance of photocatalysts is determined by three aspects: light absorption capacity, carrier separation efficiency, and carrier migration to the electrolyte/electrode interface. The carrier concentration plays an important role in increasing the rate of PC[77-79]. It can be seen in Figure 4A and B that when the temperature increases, the band gap width of the semiconductor becomes smaller, and the photoelectrode can generate more intrinsic carriers under the same photoexcitation condition. In addition, the increase in temperature can also benefit the charge transport efficiency in the electrode and promote the separation of electron-hole pairs[80-82]. In common photocatalysts, such as TiO2, BiVO4, WO3, and Fe2O3, the minority carriers are usually small polarons that are easily trapped, and an appropriate increase in temperature can activate the minority carrier transition of the semiconductor[Figure 4C][83].
Figure 4. (A) Thermally improved doping efficiency. (B) Thermally activated bound polaron hopping[83]. Copyright 2022, John Wiley & Sons, Inc. (C) A schematic illustration of the relationship between minority carrier diffusion length (LD) and particle size (LP) as a function of temperature[82]. Copyright 2016, Royal Society of Chemistry. (D) Nyquist plot of BiVO4 measured at 1.2 VRHE at different temperatures[84]. Copyright 2021, American Chemical Society.
In their study on the temperature characteristics of BiVO4 photoanodes, Zhou et al. conducted electrochemical impedance spectroscopy (EIS) to investigate the interfacial charge transfer kinetics at different temperatures[84]. As shown in Figure 4D, the Nyquist diagram at various temperatures shows the correlation between the imaginary part (ImZ(f)) and the real part (ReZ(f)). From 23 °C to 35 °C, Rs decreased by more than 40%, indicating that the electronic conductivity of BiVO4 increases with increasing temperature. At higher temperatures, the small polaron transition of BiVO4 is activated, and more intrinsic carriers are generated, which promotes the increase of photocurrent.
CLASSIFICATION OF MATERIALS FOR PHOTOTHERMAL EFFECTS
Many materials exhibit photothermal effects, and currently, five common categories of photothermal materials are applied to PC/PEC. One is metal oxides, which include a range of spinel-structured materials. The second is metal sulfides, of which the selenides within the same family also demonstrate similar photothermal properties. Third, there are metal point materials, most of which manifest plasma effects. The fourth category comprises conductive polymers, mainly polyaniline (PANI) and polypyrrole. Fifth, all kinds of carbon-based materials, such as carbon quantum dots (CQDs), graphene, carbon nanotubes (CNTs), and so on, are included. In this section, the above five photothermal materials currently applied to PC/PEC are introduced as follows. Figure 5 shows the main classification of photothermal materials.
Metal oxide
TiO2, BiVO4, and Fe2O3, which are commonly used as photocatalytic semiconductors in current research, have certain photothermal effects. Wang et al. synthesize a unique core-amorphous shell structure
Figure 6A and B lists the study of Zhou et al. on the relationship between the BiVO4 photoanode and temperature[84]. The enhancement of its photothermal effect can be attributed to two factors. First, concerning the reversible aspect, small polarons in BiVO4 are activated to promote the separation and transport of electron holes. Second, in terms of irreversibility, at high temperatures, the BiVO4 photoanode interacts with a hole scavenger to form a stepped amorphous layer on the surface, which improves the separation of charge carriers.
Figure 6. (A) Schematic diagram of improvement of charge transfer with temperature increase. (B) The change of photocurrent density of BiVO4 with temperature[84]. Copyright 2021, American Chemical Society. (C) Photocurrent density-voltage (J-V) characteristics of Si-doped α-Fe2O3 photoelectrodes at different temperatures[86]. Copyright 2014, Elsevier Ltd.
Dias et al. find that the initial potential of Si-mixed Fe2O3 changes with the increase of temperature, and the optimal initial potential is reached at 45 °C[86]. Ye et al. studied the effect of temperature on Ti-mixed
In addition to the photothermal effect of the photocatalyst itself, cobalt oxide can make the temperature rise rapidly under infrared irradiation in the loaded mixed metal oxide. He et al. place the photothermal material Co3O4 as a sandwich between the BiVO4 photoanode film and the FeOOH/NiOOH electrocatalyst sheet[88]. When irradiated by 808 nm near-infrared light, the temperature of Co3O4 rapidly rose from
Figure 7. (A) Principle of the SPECM setup. (B) Probe current curve with temperature. (C) Rate constants (Keff) of composite photoanodes at different temperatures[89]. Copyright 2021, Academic Press Inc Elsevier Sci. (D) The change of CV curves for the NCO electrode under NIR on and NIR off conditions. (E) EIS-derived Bode plots of NCO. (F) EIS-derived Bode plots of NCO-NIR[91]. Copyright 2023, Elsevier Sci.
Some metal oxides with spinel structures also have photothermal properties and are used in photocatalytic hybrid materials. He et al. studied spinel-type MCo2O4 oxide (M = Ni, Mn, Zn, Cu, and Fe) materials and designed NiCo2O4 (NCO)/BiVO4 photoanodes[90]. With the help of photothermal effects, the photocurrent density of the photoanode was 6.20 mA cm-2 at 1.23 V reversible hydrogen electrodes. The oxides of spinel structures absorb light and heat energy under infrared irradiation by virtue of their photothermal properties. The band bending at the interface between photothermal catalysts and photocatalysts is increased, which is conducive to the transfer of holes to MCo2O4.
Huang et al. use spinel oxide NCO as a photothermal material to assist TiO2 photoanodes to enhance their PEC performance[91]. NCO/TiO2 obtained a photocurrent of 2.34 mA cm-2 at 1.23 VRHE. The dynamic oxidation of Ni2+ in NCO catalysts was tracked by cyclic voltammetry (CV). As shown in Figure 7D, the oxidation peak of Ni2+ to Ni3+ transformation is between 1.3-1.4 V, and under the irradiation of near-infrared light, the oxidation peak potential continues to move downward. The results indicate that the photothermal conversion can promote the pre-oxidation of Ni2+, which is conducive to the subsequent formation and catalytic reaction of Ni-OOH. In addition, as analyzed by EIS, NCO showed an earlier transition peak at a lower potential of 1.45 VRHE under near-infrared light, which may be due to the photothermal effect that promotes faster deprotonation of *OOH [Figure 7E and F].
Hu et al. deposit ZnFe2O4 nanoparticles (NPs) on the surface of Fe2O3 and the charged small poles generated by the photothermal effect of ZnFe2O4 combined with the positively charged oxygen vacancy[92]. The synergistic effect between the photothermal effect and the oxygen vacancy improved the performance of PEC.
As the first reported photothermal material in PEC, the current research mainly focuses on the oxides containing Co and Fe elements, whose advantages are relatively low price, and as a semiconductor material itself, it can improve the performance of PEC even without heating.
Metal sulfide and metal selenide
Sulfides also have a certain photothermal effect, and the most common sulfides are mainly Cu2S, Bi2S3, MoS2, and so on[93]. Zhang et al. constructed Cu2S/Fe2O3 heterojunction photocatalysts and improved their PEC performance by utilizing the heterojunction and photothermal effects[94]. The formation of S-O bonds at the heterogeneous interface of Cu2S and Fe2O3 makes contact tighter and reduces the interface contact resistance, thus promoting charge transfer and improving stability. In addition, Cu2S itself has high photothermal properties, and its photothermal effect makes the heterojunction electrode reach a local high temperature under light, which is conducive to accelerating the OER rate[95].
Zhao et al. deposit the narrow band gap semiconductor Bi2S3 on the surface of WO3 nanosheets[96]. Using the photothermal conversion characteristics of Bi2S3, the photocurrent density of Bi2S3/WO3 composite photoanodes to the reversible reference electrode reaches 4.05 mA cm-2 at 1.23 V (VRHE). An EIS Nyquist test was performed on the material to analyze its photothermal effect. The fitting values extracted from the Nyquist diagram are listed in Table 1. The Rbulk value of PI-WO3/Bi2S3 (25.97 Ω) is smaller than that of
Fitting results of Rs, Rbulk, and Rint for different samples by ZVIEW[96]
Photoanodes | Rs (Ω) | Rbulk (Ω) | Rint (Ω) |
WO3 | 25.60 | 57.58 | 5,713 |
PI- WO3 | 25.36 | 53.68 | 4,890 |
Bi2S3/WO3 | 25.62 | 43.61 | 2,905 |
PI- Bi2S3/WO3 | 25.20 | 25.97 | 1,898 |
In addition, some ternary sulfides, CdSeS, ZnInS, and ZnCdS, which have similar spinel structures, have also been applied to photothermal PEC processes[97-99].
As a congener of sulfides, selenides have similar properties, but there is currently limited research on metal selenides used in photothermal applications. Li et al. prepared MoSe2-CDs-ZnO (M2CZ2), in which MoSe2 nanosheets strongly absorb light at the full spectrum (from UV to near-infrared) and can be used as the main photothermal materials[100]. The hydrogen production of M2CZ2 was investigated under the condition of a water bath. After heating for 4 h, the H2 precipitation of M2CZ2 reached 158.6 μmol cm-2. As shown in Figure 8A, after 2 min irradiation, M2CZ2 increased by about 32 °C, indicating a significant temperature rise, while the control group only increased by 15 °C. Figure 8B shows the temperature changes of different electrodes in electrolytes under the irradiation of 300 W Xe lamps. M2CZ2 increases by 20 °C, which proves that it can also significantly increase temperature in PEC systems. The reaction hydrogen production process and thermal imaging diagram are shown in Figure 8C and D.
Figure 8. (A) Photocatalytic hydrogen production at a photoelectrode with water bath heating at 0 V (vs. Ag/AgCl). (B) Photocatalytic hydrogen production at a photoelectrode without water bath heating at 0 V (vs. Ag/AgCl). (C) H2 generation on Pt counter electrodes with light irradiation. (D) Infrared thermal images of the CdS-ZnO-Au-PWE (a-d) and M2CZ2 (e-h) under different irradiation times of 300 W Xe lamps[100]. Copyright 2021, Elsevier Ltd.
As S and Se are in the same family as O, the properties of sulfides and selenides themselves have many similarities with oxides. However, due to the fact that the synthesis of sulfide (selenide) itself produces toxic gases, such as hydrogen sulfide, and the cost is relatively higher, there are few literature studies on these two types of materials.
Metal nanodots
Among many photothermal materials, metal nanodot materials have their unique photothermal properties, that is, the local surface plasmon resonance effect (LSPR) of metal NPs[101,102]. The size of the metal NPs is smaller than the wavelength of the incident light, and the free electrons on the surface of the conductive material are excited and oscillate[103]. When the electron vibration frequency matches the incident light frequency, the metal NPs will produce strong absorption of the photon energy, resulting in resonance effects, which will stimulate the production of hot electrons [Figure 9][103-105].
Figure 9. Four energy transfer mechanisms from a plasmonic NP to an n-type semiconductor to drive water oxidation: (A) light scattering, (B) hot electron injection, (C) light concentration, and (D) plasmon-induced resonance energy transfer (PIRET)[104]. Copyright 2015, American Chemical Society.
Plasma materials require good chemical stability and high stability. Among them, Au NPs, as plasma materials, have been widely studied for PEC[106,107]. Tang et al. deposited NiCoOx electrocatalytic layers on the surface of Au NPs[108]. Due to the LSPR and photothermal effect of plasma Au NPs, the current density of NiCoOx/Au anode increased by 7.01 mA cm-2 under light. Figure 10A and B shows optical simulations of Au NPs and NiCoOx separated Au NPs at an excitation wavelength of 550 nm. The edge of the Au NPs in the two images forms a strong spatial non-uniform oscillating electric field, which is a characteristic of the LSPR effect. Agarwal et al. use a silicon material as the inner core of the Au-loaded plasma film (Au-coated Si nanowire)[109]. The use of a semiconductor metal nanowire cavity to enhance the plasma properties of the Au NPs results in the resonance of intense heat [Figure 10C]. Under light conditions, the temperature in the nanowire cavity reaches ~1,000 K, which increases the H2 formation rate of ethanol photoreforming reactions by about 40%. Zhang et al. deposited 20 nm Au NPs on the nanotube array of TiO2 and used the hot electrons generated by the LSPR effect of Au to inject the conduction band of TiO2, resulting in a photocurrent density of about 150 μA cm-2 under visible light[106].
Figure 10. (A) Spatial normalized distribution of electric fields of gold nanoparticles (top) and NiCoOx-separated gold nanoparticles (bottom). (B) Spatial distribution of electric field intensity inside the cavity under TM excitation for 10 nm Au-coated Si nanowire at
The cost of Au is expensive, and metals Ag, Cu, Fe, Ni, Bi, and other NPs are also used as cost-effective materials[110-113]. Song et al. prepare Cu/TiO2 NPs, and the introduction of 3-6 nm Cu NPs significantly improved the photocatalytic hydrogen production rate of TiO2[114]. The photothermal effect of Cu NPs causes the local temperature of the catalyst surface to increase, which significantly reduces the activation energy of the reaction and improves the charge separation efficiency [Figure 10D]. Li et al. use a chemical reduction method to hybridize Cu NPs with TiO2in situ[115]. Under the display of an infrared thermal imager, the temperature of Cu/TiO2 composites can reach about 80 °C after 2 min of illumination, while the temperature rise of TiO2 is not obvious. In the test of hydrogen production, the hydrogen production of Cu/TiO2 gradually rises with the increase of temperature, and the hydrogen production rate is as high as 24,160.69 μmol g-1 h-1 at 100 °C. This provides a low-cost and high-efficiency preparation method of noble-free photocatalysts for constructing photocatalytic materials. Subramanyam et al. add Bi NPs to the
The photothermal effect of metal nanodots is largely derived from the plasmon resonance effect. At present, the application reports of metal nanodots are mainly concentrated in the field of PC. Among them, the photothermal effect of Au and Ag is better, but the corresponding cost and synthesis conditions are also elevated. At present, the research of Cu nanodots is more popular, and the investigation of other common metal nanodots needs to be further explored.
Conductive polymer
As an organic conductive material, conductive polymers are mainly based on hole transport, and it has been proved by experiments that they also have photothermal conversion effects, which can be applied in photothermal PEC[117].
Zhao et al. constructed Co-Pi/PANI/BiVO4 composite photoanodes with PANI and cobalt phosphate
Figure 11. (A) Temperature-dependent photocurrent-voltage curves of the BiVO4 photoanode. (B) Temperature-time plots of BiVO4 and PANI/BiVO4 with NIR light[118]. Copyright 2020, Royal Society of Chemistry. (C) Schematic illustration of the band energy alignment of CIS/Ni-PPy + NIR photoanodes[119]. Copyright 2022, John Wiley & Sons, Inc.
Xu et al. propose to use the conjugated polymer polypyridine (PPy) with high conductivity and good photothermal effects as a multifunctional surface modifier for the photoelectrochemical (PEC) water decomposition of terene metal sulfides (CdIn2S4, CIS)[119]. The principle of near-infrared photoanodes applied to PEC water cracking is shown in Figure 11C. The introduction of Ni further accelerates the surface reaction kinetics, including the reduction of charge transfer resistance and the negative displacement of the plane band potential. In addition, PPy exhibits photothermal properties under near-infrared radiation, which can be targeted to improve the surface temperature of the photoanode material.
At present, the research literature on photothermal materials of conductive polymers is the scarcest compared with other types of materials, and the main field is focused on PEC. Conductive polymers inherently possess good electrical conductivity and light absorption effects, but the challenge lies in effectively combining conductive polymers with photoelectric materials. Therefore, they hold substantial development prospects in the future development of photothermal materials.
Carbon-based materials
In many studies, carbon-based materials are often used as carriers of electrocatalysts because of their large surface area and excellent charge mobility. The carbon-based material itself has a darker color, and it also has a good application prospect in the light absorption of photothermal conversion[120-122].
CQDs, as typical carbon-based photothermal materials, can effectively convert the energy of near-infrared light into local heat and are the most widely used photothermal materials in carbon-based materials[123].
Cai et al. prepare (Ti, Zn) -Fe2O3@Ti -Fe2O3@C&Co-Pi by means of heteroatomic mixing, in which CQDs are attached to Fe2O3 rods in the form of anchor points[125]. CQDs produce local thermal effects under near-infrared irradiation; the photocurrent density increases to 1.02 mA cm2, and the initial potential shifts by 0.87 V. The photothermal effect increases the contact potential difference and band bending at the semiconductor-electrolyte interface [Figure 12].
Figure 12. (A) The structure diagram of (Ti, Zn) -Fe2O3@Ti -Fe2O3@C&Co-Pi. (B) The temperature variation of the sample under 10 W illumination. (C) The band alignment of the sample under AM 1.5 and NIR illumination[125]. Copyright 2023, Elsevier Ltd.
As a two-dimensional conductive material, the excellent electrical properties of graphene find frequent applications in photoelectric material composites[126]. Wu et al. synthesize rGO/Na2Ti3O7 nanospheres by a hydrothermal method [Figure 13A][127]. The coupling of layered Na2Ti3O7 and rGO significantly improved the PEC properties under visible light irradiation. Under light irradiation, the rGO/Na2Ti3O7 microsphere can act as a microheater, and the local temperature is significantly increased, making it much higher than the average solution temperature. This local thermal effect can effectively absorb the reactants and significantly accelerate the reaction kinetics. In addition, the electrons on the rGO sheet can gain extra energy to move quickly, inhibiting the recombination of electron holes.
Figure 13. (A) Schematic diagram of the growth mechanism of Na2Ti3O7 and rGO/Na2Ti3O7 microspheres[127]. Copyright 2020, American Chemical Society. (B) Hydrogen evolution rate over melamine (M-CN) via the TPC (80 °C), PC and TC (80 °C) pathways. (C) Hydrogen evolution rate over cyanamide (C-CN) via the TPC, PC and TC pathways[128]. Copyright 2023, Elsevier Ltd.
In Figure 13B and C, Fang et al. synthesize g-C3N4 materials by a hard template method and study the hydrogen production rate of g-C3N4 under three ways of photothermal catalysis, PC, and thermal catalysis[128]. At 60 °C, the photothermal synergistic hydrogen production rate reaches 1,932.9 μmol g-1 h-1, which is much higher than that of thermal catalysis or PC alone. With the increase of temperature, the thermal catalytic performance of C3N4 is improved more obviously, which shows that C3N4 has a good photothermal conversion performance.
In addition, the photothermal properties of carbon hemispheres, three-dimensional CNTs, and carbonized melamine foam (C-MF) have also been reported [129,130].
Other photothermal materials
In recent years, a variety of photothermal materials have appeared, such as organic matter, MXenes, elemental S, etc., which can improve the photocatalytic performance by increasing the temperature of the photoelectrode.
As two-dimensional metal carbide materials, MXenes have many similarities with graphene in structures and properties. Under infrared irradiation, MXene materials can generate a lot of heat and increase the surface temperature of the photoanode. Xie et al. graft MXene nanosheets as surface modifiers onto ZnO nanorods[131]. Under light, the temperature of MXene nanosheets rapidly increased from 17.8 °C to 35.7 °C [Figure 14A].
Figure 14. (A) Time-dependent temperature curves of the electrolyte ZnO/MXene nanorod arrays irradiated by 808 nm NIR light[131]. Copyright 2022, American Chemical Society. (B) The UPS spectra of NiFeOxHy/CC and S-NiFeOxHy/CC. (C) The UV-vis-NIR absorption spectrum of S-NiFeOxHy/CC[132]. Copyright 2023, Elsevier Ltd.
Zhang et al. constructed sulfur-mixed iron nickel-metal hydride oxide (S-NiFeOxHy/CC) on carbon cloth[132]. As shown in Figure 14B, UPS tests show that the valence band of S-NiFeOxHy/CC shifts towards Fermi level when sulfur is mixed, which makes S-NiFeOxHy/CC have stronger light absorption performance for the full spectrum of sunlight. The surface temperature of S-NiFeOxHy/CC increases rapidly under light, up to 82.6 °C [Figure 14C].
In addition, there are also researchers to develop various types of photothermal materials to harness synergistic effects.
Zhang et al. prepare a snowflake Cu2S/MoS2/Pt material by a hydrothermal method and electrodeposition[133]. Under infrared induction, the photothermal effect of MoS2 can generate a larger photocurrent by increasing the electron transfer speed and interface electrochemical reaction, and the LSPR effect of Pt NPs further stabilizes the photothermal properties of the material. At the same time, due to the special geometric and electronic structure of Cu2S, it has a positive effect on hydrogen production by PEC.
Cao et al. constructed CQDs/Au/TiO2 nanorod array composite photoanodes[134]. The electric field of CQDs/Au/TiO2 photoanodes was simulated by a finite element method. Due to the SPR effect, the electric field near Au is obviously enhanced. The coupling between Au and TiO2 promotes the transfer of hot electrons to the semiconductor through the metal-semiconductor interface. The electric field inside CQDs is the smallest in the whole region, which plays the role of hole heat collection. The synergistic effect of Au and CQDs not only stimulates the small polaron transport activity of semiconductor TiO2 but also accelerates the charge transfer and surface reaction from the aspects of kinetics and thermodynamics. The H2 precipitation rate of CQDs/Au/TiO2 photoanodes is five times that of blank TiO2.
By electrodeposition and CV, Zhao et al. load Au NPs and rGO onto TiO2 nanotube arrays, respectively, to prepare Au/rGO/TiO2 photoelectrodes[135]. COMSOL is used to simulate the temperature field of the photoelectrode. As shown in Figure 15A and B, due to the high thermal conductivity of rGO, the heat transfer of Au NPs to TiO2 can be accelerated, so Au-rGO/TiO2 can more fully transfer heat from the top of the nanoring to the nanotube. The synergistic effect between the thermal plasma effect of Au NPs and the excellent heat transfer performance of graphene oxide was confirmed.
Figure 15. (A) Thermal imaging of TiO2-based photoelectrodes under AM 1.5 irradiation change over time. (B) Steady-state temperature simulation of Au NPs as nano-heat sources (COMSOL)[135]. Copyright 2023, Elsevier Ltd.
APPLICATION OF PHOTOTHERMAL MATERIALS
The research of photothermal materials is not only limited to the mechanism of photothermal generation of the material itself but also fully applied to its photothermal properties in all directions. Through the combination of PC, solar cells, etc., the performance of photothermal materials can be realized[136-138]. The energy utilization rate can be improved, and the product conversion rate can be improved. Figure 16 shows the application areas of different photothermal materials.
PC and photothermal effect
Photothermal catalytic water decomposition
For photocatalytic water decomposition, the thermal energy converted by the full spectrum of sunlight can effectively improve the catalytic performance. For photocatalytic water decomposition, the thermal energy converted by the full spectrum of sunlight can effectively improve the catalytic performance. Table 2 lists the hydrogen production performance of TiO2 supported by different photothermal materials at elevated temperatures.
Hydrogen production rate of photothermal catalytic hydrogen evolution material under thermal effect
Hu et al. prepared rGO-modified Cu2O-supported TiO2 photocatalysts and applied them to photothermal synergistic catalytic hydrogen production[142]. Under the condition of PC, the hydrogen production rate reached 178 μmol·h-1 at 90 °C, which is about 4.7 times the sum of photocatalytic and thermocatalytic hydrogen evolution activities (38 μmol·h-1) at 25 °C. It can be seen that the amount of hydrogen evolution under photothermal coupling catalysis is much higher than that under PC or thermocatalysis. According to the analysis of solar spectral light absorption, TiO2, Cu2O, and rGO in the composite material represent the utilization of UV light, visible light, and infrared light, respectively, achieving gradient utilization. The photothermal effect produced by graphene between semiconductors can accelerate the rate of electron and hole transfer on the catalyst surface.
Zhang et al. also use the gradient absorption of solar energy to co-modify In2O3 with transition metals Fe and Cu[143]. Fe doping widens the radiation response range of the intrinsic part of the semiconductor, while Cu doping is beneficial to the absorption of visible infrared light and produces photothermal synergies. As shown in Figure 17A, the photo-driven photothermal catalytic reaction system (PDS) is built with quartz, and the system energy uses full-spectrum xenon lamps. The catalyst film was placed on the quartz scaffold, and the reaction temperature was maintained by the thermal effect generated by the light catalyst film. The DFT calculation of the reaction process is also carried out, and the results show that the increase of temperature can directly affect the chemical reaction by reducing the energy barrier of RDS.
Figure 17. (A) Schematic diagram of a photo-driven photothermal catalytic reaction system (PDS)[143]. Copyright 2023, American Chemical Society. (B) Physical images of C-MF at different locations in solution. (C) Schematic diagram and thermographic image of C-MF/CIZS@Ru in solution illuminated at different positions for 1 h[144]. Copyright 2022, American Chemical Society.
Ding et al. coated CIZS@Ru photocatalysts on the surface of a C-MF net[144]. In the photocatalyst, C-MF absorbs vision-infrared light, while the photocatalyst captures UV-visible light. A spatially separated photothermal coupling photocatalytic reaction system was designed. The whole device can float on the water surface, reducing the refraction loss of light through the water and the transmission resistance of hydrogen [Figure 17B and C]. The distribution of the photocatalyst on the surface of the photothermal material reduces the local heating distance and helps to improve the influence of local heat on the photocatalytic activity. Driven by the photothermal effect, the hydrogen evolution performance of the material reached 603 mmol h-1 m-2.
Photocatalysis and water vapor
For the water decomposition process, the increase in temperature will promote the conversion of water into water vapor, increasing the catalytic contact surface.
Han et al. prepare the g-C3N4 (CW-HCN) system by growing spherical g-C3N4 (HCN) on carbonized wood (CW) by hydrothermal methods[145]. The H2 precipitation rate reached 2,700.18 μmol m-2 h-1 under sunlight. As shown in Figure 18A, CW acts as the growth base for the g-C3N4 photocatalyst and also acts as a steam generator. The carbonization layer uses visible and near-infrared light in the solar spectrum to produce steam, while the UV light and part of the visible light are absorbed by g-C3N4 to produce electron-hole pairs for the decomposition of water. The combination of photoheat and PC greatly expands the utilization of solar photons.
Figure 18. (A) Photo of the photothermal-photocatalytic H2 evolution system[145]. Copyright 2023, Academic Press Inc Elsevier Sci. (B) The Schematic of the utilization of sunlight and water splitting performance in liquid-solid and gas-solid systems with different catalyst dispersion states under high light intensity[146]. Copyright 2023, John Wiley & Sons, Inc. (C) Schematic diagram of a thermocouple-controlled photocatalytic CO2 reduction device[147]. Copyright 2021, American Chemical Society.
Li et al. design K-SrTiO3 catalysts loaded with TiN silica wool at the water-air interface[146]. The thermal effect is used to convert liquid water into water vapor, thereby reducing the reaction-free energy of the catalyst and improving the permeability of the catalytic product. The photothermal catalytic hydrogen production efficiency can reach 275.46 mmol m-2 h-1, which is more than twice the decomposition efficiency of liquid water [Figure 18B].
Photothermal catalysis and CO2 reduction
Photothermal catalysis is used to reduce CO2 and convert CO2 into fuel through photothermal catalysis.
Photothermal catalysis and organic degradation
PC is a common method used in organic matter degradation, and the efficiency of photocatalytic pollution degradation can be further improved by using the photothermal effect[148,149]. Huang et al. introduced oxygen vacancies into BiOI nanosheets (named BiOI-8) with low concentration nitric acids and applied them to photocatalytic degradation of formaldehyde[150]. As shown in Figure 19A, the photocatalytic performance of BiOI-8 nanosheets improved with the increase of temperature. The catalytic activity of BiOI-8 nanosheets at 60 °C was 20% higher than that at room temperature. The BiOI-8 nanosheets were tested in an industrial-grade photocatalytic unit [Figure 19B]. Huang et al. prepared CeO2/CeN photocatalysts[151]. The prepared CeO2/CeN composites showed higher photothermal catalytic performance than the original CeO2 in removing organic pollutants. The enhancement of photothermal catalytic activity is due to the formation of the phase interface boundary of effective photothermal catalytic degradation due to the introduction of CeN [Figure 19C and D].
Figure 19. (A) Photocatalytic degradation of HCHO at different temperatures at 45 min. (B) Photograph of large-scale photocatalytic devices[150]. Copyright 2018, Elsevier Inc. (C) Photo-thermocatalytic performance of pollutant degradation. (D) Representation of the formation of CeO2/CeN nanorods[151]. Copyright 2018, Royal Society of Chemistry.
PEC and photothermal effect
PEC and photothermal effect
In the application of photothermal materials in PEC water decomposition, there are more reports on the enhancement of photothermal effects by applying infrared radiation. As shown in Table 3, the photocurrent density of PEC of different materials is significantly improved with its addition.
Compare the performance of PEC with or without NIR
Photoanode | Photothermal effector materials | Photocurrent density (1.23 VRHE) | Photocurrent density with NIR (1.23 VRHE) | Reference |
Au/Ni/ITO/BiVO4@CoPi | Ni | 3.28 mA cm-2 | 5.19 mA cm-2 | [83] |
NiOOH/FeOOH/Co3O4/BiVO4 | Co3O4 | 4.49 mA cm-2 | 6.34 mA cm-2 | [88] |
Bi2S3/WO3 | Bi2S3 | 3.60 mA cm-2 | 4.60 mA cm-2 | [96] |
PANI/BiVO4 | PANI | 3.01 mA cm-2 | 4.05 mA cm-2 | [118] |
CIS/Ni-PPy | PPy | 3.75 mA cm-2 | 6.07 mA cm-2 | [119] |
Co-Pi/CQDs/Fe2O3/TiO2 | CQDs | 1.65 mA cm-2 | 3.00 mA cm-2 | [124] |
Xiong et al. built CoCr2O4/g-C3N4 and used the strong absorption of CoCr2O4 NPs in the near-infrared region to improve the temperature of the reaction center to improve the performance of PEC[152]. The simple CoCr2O4 NPs did not show photocatalytic activity of PEC hydrogen production, but the average hydrogen production rate under UV-vis-IR was as high as 1,525.1 μmol g-1 h-1, which was four times higher than that of pure g-C3N4.
Lin et al. load NCO nanomedles with nickel foam (NF) to promote their OER properties through infrared thermal effects[153]. The local thermal effect of infrared radiation on the catalyst increases the surface temperature of NCO, which proves that the decrease of electrochemical activation energy caused by infrared thermal effects is the reason for the increase of OER activity. It can be seen from the linear sweep volt (LSV) curve [Figure 20A] that NF/NCO exhibits significantly enhanced OER activity under continuous infrared irradiation. After infrared irradiation, the surface temperature of NF/NCO rose sharply and reached 48.1 °C within 15 s. The nanoneedle arrays of NCO further improve infrared absorption by providing a larger specific surface area and reduced infrared diffuse reflection. The reaction devices are shown in Figure 20B and C.
Figure 20. (A) LSV curves obtained on the NF/NiCo2O4. (B) Structure of jacketed glass electrochemical cell for PEC performance measurement. (C) NiCo2O4 (NF/NiCo2O4) electrodes supported by nickel foam with and without NIR[153]. Copyright 2023, Academic Press Inc Elsevier Sci.
Dong et al. design a co-modified N,Ni-BVO/N-TNA photoanode with N and Ni double-point defects on the basis of BiVO4/TiO2 heterojunction photocatalysts[154]. Through co-doping, oxygen vacancies are formed, greatly improving the photothermal effect of the infrared drive, which makes the maximum temperature of the lifting solution rise to 48.6 °C. The increase of solution temperature also decreases the interfacial resistance of the heterojunction, leading to faster electron transport.
Thermal effect of PEC combined with perovskite batteries
Perovskite is one of the most widely used materials in solar cells. PEC can be realized by its effect of light absorption and light heat transfer[155].
Tang et al. design a composite photoelectrode (Au/Ni/ITO/BiVO4@CoPi) with both photothermal and photoelectric conversion[83]. As shown in Figure 21A, bismuth vanadate and perovskite cells absorb sunlight in the bands of 300-505 nm and 505-850 nm to generate sufficient water decomposition photovoltage and photocurrent. The 850-2,000 nm (infrared light region) nanometer metal nickel layer is used to generate heat to promote the photogenic charge collection process and water decomposition reaction rate of the electrode. The system can increase the electrolyte temperature from 293.15 K to 328.15 K by self-drive and achieve 4.28 mA cm-2 light-driven water and light decomposition current. The photothermal substrate
Figure 21. (A) L-type photothermal PEC-PV device schematic[83]. Copyright 2022, John Wiley & Sons, Inc. (B) Cross-section SEM image of the semitransparent PSC. (C) The integrated ST-PSC+EC system for water splitting[129]. Copyright 2023, Elsevier Sci. (D) Structure and schematic diagram of the solar desalination system[157]. Copyright 2023, Elsevier Sci.
Wang et al. deposit NiFe layer double hydroxide (NiFe LDH) on a three-dimensional CNT scaffold[129]. A water decomposition system was constructed by connecting a CNTs@NiFe LDH//Pt/C electrochemical cell (EC) with a semitransparent perovskite solar cell (ST-PSC) micromodule in series [Figure 21B]. Infrared radiation can pass through the translucent PSCs, introducing a photothermal effect on the EC electrode, thus making better use of the full solar spectrum. With the aid of the photothermal effect, the STH efficiency of the tandem system is improved by 12%. The reaction device is shown in Figure 21C.
Photothermal effect of PEC and seawater desalination
In the earth's water resources, the effective use of seawater can effectively achieve environmental energy saving and water resource regeneration[156].
Cai et al. use the photovoltaic and photothermal coupling effects of GeSe-based photoelectrodes to be applied in solar desalination[157]. When combined under the irradiation of AM 1.5 G, it was found that the temperature of the GeSe system was significantly higher than that of the solution (up to 323 K) because GeSe converted part of the absorbed visible light into heat. Therefore, thermoelectric devices (TD) with GeSe bases can be introduced to make full use of the full spectrum of solar energy. As shown in Figure 21D, the TD-GeSe-based solar desalination battery is integrated and coupled with the GeSe-based photocathode to form a TD-GeSe-based solar desalination battery and placed between the desalination chamber and the cooling chamber to achieve sustainable and efficient seawater desalination. This method effectively converts natural seawater to seawater close to fresh water, reducing the total dissolved solid solution and salinity of the original solution from 29 g L-1 and 27 ppm to about 1.0 g L-1 and 0.7 ppm, respectively.
Photothermal effect and bionic structure of PEC
The research of PEC is nothing more than an artificial way to achieve biological photosynthesis, and the construction of biomimetic structure materials applied to PEC can effectively improve its photoelectric catalytic and photothermal catalytic performance[158].
Inspired by the photosynthesis of plant leaves, Zhou et al. load the photothermal MoS2/FeCoNiS nanotubes onto porous NF (PNF)[159]. As shown in Figure 22A, PNF and MoS2/FeCoNiS resemble the structure and epidermis of leaf tissue, respectively. The stomatal structure of PNF promotes bubble transport in OER, while MoS2/FeCoNiS increases light absorption and photothermal conversion. As the electrolyte temperature increases from 25 °C to 55 °C, the OER performance of MoS2/FeCoNiS-NT@PNF improves significantly. With the help of bionic structures and photothermal effect, the MoS2/FeCoNiS-NT@PNF electrode achieves a current density of 50 mA cm-2 at 1.44 V (vs. RHE).
Zhang et al. prepare a MoS2/Ni3S2-coated carbon nanowires (CA) composite (MoS2/Ni3S2@CA) by tracking the sun to raise the temperature from a natural sunflower head[160]. MoS2/Ni3S2@CA has a honeycomb structure similar to the microstructure of sunflower petals. The carbon nanowire substrate with three-dimensional multistage pore structures can increase the active sites of catalyst nanosheets and accelerate the transfer process of bubbles and electrolytes. The biomimetic sunflower micro-nanostructures are able to better capture sunlight and efficiently and quickly convert light into heat. The current density of
CONCLUSIONS
In this paper, the basic principle of the photothermal effect is briefly introduced, and several kinds of photothermal materials widely reported at present are reviewed. The reason why the photothermal effect improves the photocatalytic performance is analyzed from the perspective of physical models and principle analysis. The introduction of thermal effects can improve the thermodynamic and kinetic effects of the catalytic reaction itself. At the same time, most photothermal materials themselves produce more excitons due to the increase of temperature. The characteristics of different types of photothermal materials are reviewed, and their respective advantages are analyzed. Finally, the application of photothermal effects in PC and PEC in reduction, oxidation, and other specific fields is summarized. Whether it is photothermal catalysis or photothermoelectric catalysis, it is an innovation of traditional photocatalytic technology. The addition of thermal effects is not a simple one-plus-one process but rather a coordination of the two effects from the mechanism to achieve improvement. From the perspective of long-term development, it is a feasible industrial practice to improve energy utilization and reduce losses through a variety of energy cooperative coupling.
Although the development of PC/PEC photothermal materials is expected in the future, there are still some challenges:
1. Photothermal catalysts: currently, precious metal-supported photothermal catalysts are the most widely used in current research because of their high catalytic activity. However, their high cost limits their application and promotion. In addition, challenges related to poor light absorption and conversion ability, low activity, and low stability of photothermal materials persist as fundamental issues in the research and development of photothermal materials. In summary, the development of low-cost and high-activity materials is the main direction of further research.
2. Combination of photothermal materials and photocatalytic materials: in the current reports on the application of photothermal materials to PC, most of the photothermal materials and photocatalysts are composites with a layered structure. The way of their mutual connection and action is generally through physical connection or hydrogen bond connection, and the combination degree of the two needs to be further improved. If photothermal and photocatalytic materials can be combined at the nanoscale, it will help to improve the energy and electron conduction between these materials so as to achieve higher conversion efficiency.
3. Photothermal reactor: the current photothermal reaction system will build more devices through external connections to achieve higher conversion efficiency. However, this approach seems to go against the original intention of PC itself to achieve energy conversion in a clean and efficient way. Therefore, for future photothermal reactors, it is recommended to utilize sunlight as the only light source and harness its energy to achieve catalytic effects.
4. Development of the photothermal materials industry: many factors influence the performance of photothermal catalysis at present, and its effectiveness is determined by different conditions, such as light intensity, light band, bias pressure, and reactor temperature. However, the current research field has yet to establish a standardized evaluation system for this series of photocatalysts, hindering the development of an industrial evaluation framework. In addition, the monitoring and control of various parameters of the reaction process have not been able to determine clear process parameters. There is still a long way to go for the large-scale industrial promotion of photothermal materials.
DECLARATIONS
Authors’ contributions
Characterizing and writing the original draft: Zhang J
Review and supervision: Ye KH, Shi J
Editing the manuscript: Tang T, Yang R, Wang G
Availability of data and materials
Not applicable.
Financial support and sponsorship
None.
Conflicts of interest
All authors declared that there are no conflicts of interest.
Ethical approval and consent to participate
Not applicable.
Consent for publication
Not applicable.
Copyright
© The Author(s) 2024.
REFERENCES
1. Fares RL, Webber ME. The impacts of storing solar energy in the home to reduce reliance on the utility. Nat Energy 2017;2:17001.
2. Kammen DM, Sunter DA. City-integrated renewable energy for urban sustainability. Science 2016;352:922-8.
3. Lelieveld J, Evans JS, Fnais M, Giannadaki D, Pozzer A. The contribution of outdoor air pollution sources to premature mortality on a global scale. Nature 2015;525:367-71.
4. Dawood F, Anda M, Shafiullah G. Hydrogen production for energy: an overview. Int J Hydrog Energy 2020;45:3847-69.
5. Tee SY, Win KY, Teo WS, et al. Recent progress in energy-driven water splitting. Adv Sci 2017;4:1600337.
6. Zou X, Zhang Y. Noble metal-free hydrogen evolution catalysts for water splitting. Chem Soc Rev 2015;44:5148-80.
7. Muradov N. Low to near-zero CO2 production of hydrogen from fossil fuels: status and perspectives. Int J Hydrog Energy 2017;42:14058-88.
8. Ran J, Jaroniec M, Qiao SZ. Cocatalysts in semiconductor-based photocatalytic CO2 reduction: achievements, challenges, and opportunities. Adv Mater 2018;30:1704649.
9. Abbott D. Keeping the energy debate clean: how do we supply the world's energy needs? Proc IEEE 2010;98:42-66.
10. Thalluri SM, Bai L, Lv C, Huang Z, Hu X, Liu L. Strategies for semiconductor/electrocatalyst coupling toward solar-driven water splitting. Adv Sci 2020;7:1902102.
11. Fujishima A, Honda K. Electrochemical photolysis of water at a semiconductor electrode. Nature 1972;238:37-8.
12. Butburee T, Bai Y, Wang H, et al. 2D Porous TiO2 single-crystalline nanostructure demonstrating high photo-electrochemical water splitting performance. Adv Mater 2018;30:e1705666.
13. Liu X, Wang F, Wang Q. Nanostructure-based WO3 photoanodes for photoelectrochemical water splitting. Phys Chem Chem Phys 2012;14:7894-911.
14. Chen D, Xie Z, Tong Y, Huang Y. Review on BiVO4-based photoanodes for photoelectrochemical water oxidation: the main influencing factors. Energy Fuels 2022;36:9932-49.
15. Hsu YK, Chen YC, Lin YG. Novel ZnO/Fe2O3 core-shell nanowires for photoelectrochemical water splitting. ACS Appl Mater Interfaces 2015;7:14157-62.
16. Zhang Y, Pan D, Tao Y, et al. Photoelectrocatalytic reduction of CO2 to syngas via SnOx-enhanced Cu2O nanowires photocathodes. Adv Funct Materials 2022;32:2109600.
17. Pan L, Muhammad T, Ma L, et al. MOF-derived C-doped ZnO prepared via a two-step calcination for efficient photocatalysis. Appl Catal B 2016;189:181-91.
18. Wang L, Shi X, Jia Y, Cheng H, Wang L, Wang Q. Recent advances in bismuth vanadate-based photocatalysts for photoelectrochemical water splitting. Chin Chem Lett 2021;32:1869-78.
19. Zhang J, Huang Y, Lu X, Yang J, Tong Y. Enhanced BiVO4 photoanode photoelectrochemical performance via borate treatment and a NiFeOx cocatalyst. ACS Sustain Chem Eng 2021;9:8306-14.
20. Lin F, Boettcher SW. Adaptive semiconductor/electrocatalyst junctions in water-splitting photoanodes. Nat Mater 2014;13:81-6.
21. Xie Z, Chen D, Zhai J, Huang Y, Ji H. Charge separation via synergy of homojunction and electrocatalyst in BiVO4 for photoelectrochemical water splitting. Appl Catal B 2023;334:122865.
22. Digdaya IA, Adhyaksa GWP, Trześniewski BJ, Garnett EC, Smith WA. Interfacial engineering of metal-insulator-semiconductor junctions for efficient and stable photoelectrochemical water oxidation. Nat Commun 2017;8:15968.
23. Bae D, Seger B, Vesborg PC, Hansen O, Chorkendorff I. Strategies for stable water splitting via protected photoelectrodes. Chem Soc Rev 2017;46:1933-54.
24. Chen D, Liu Z, Guo Z, Yan W, Ruan M. Decorating Cu2O photocathode with noble-metal-free Al and NiS cocatalysts for efficient photoelectrochemical water splitting by light harvesting management and charge separation design. Chem Eng J 2020;381:122655.
25. Zandi O, Hamann TW. Enhanced water splitting efficiency through selective surface state removal. J Phys Chem Lett 2014;5:1522-6.
26. Sivula K. Metal oxide photoelectrodes for solar fuel production, surface traps, and catalysis. J Phys Chem Lett 2013;4:1624-33.
27. Liu W, Liu H, Dang L, et al. Amorphous Cobalt-iron hydroxide nanosheet electrocatalyst for efficient electrochemical and photo-electrochemical oxygen evolution. Adv Funct Mater 2017;27:1603904.
28. Meng Q, Zhang B, Yang H, et al. Remarkable synergy of borate and interfacial hole transporter on BiVO4 photoanodes for photoelectrochemical water oxidation. Mater Adv 2021;2:4323-32.
29. Liang Z, Li M, Ye K, et al. Systematic engineering of BiVO4 photoanode for efficient photoelectrochemical water oxidation. Carbon Energy 2023:e413.
30. Yang J, Zhou J, Huang Y, Tong Y. Lanthanide-based dual modulation in hematite nanospindles for enhancing the photocatalytic performance. ACS Appl Nano Mater 2022;5:8557-65.
31. Ye K, Hu P, Liu K, et al. New findings for the much-promised hematite photoanodes with gradient doping and overlayer elaboration. Solar RRL 2022;6:2270061.
32. Liu J, Chen W, Sun Q, et al. Oxygen vacancies enhanced WO3/BiVO4 photoanodes modified by cobalt phosphate for efficient photoelectrochemical water splitting. ACS Appl Energy Mater 2021;4:2864-72.
33. Yang W, Prabhakar RR, Tan J, Tilley SD, Moon J. Strategies for enhancing the photocurrent, photovoltage, and stability of photoelectrodes for photoelectrochemical water splitting. Chem Soc Rev 2019;48:4979-5015.
34. Wang S, Chen P, Bai Y, Yun JH, Liu G, Wang L. New BiVO4 dual photoanodes with enriched oxygen vacancies for efficient solar-driven water splitting. Adv Mater 2018;30:e1800486.
35. Butson JD, Sharma A, Tournet J, et al. Unlocking ultra-high performance in immersed solar water splitting with optimised energetics. Adv Energy Mater 2023;13:2301793.
36. Kim H, Seo JW, Chung W, et al. Thermal effect on photoelectrochemical water splitting toward highly solar to hydrogen efficiency. ChemSusChem 2023;16:e202202017.
37. Li T, Zou Y, Liu Z. Magnetic-thermal external field activate the pyro-magnetic effect of pyroelectric crystal (NaNbO3) to build a promising multi-field coupling-assisted photoelectrochemical water splitting system. Appl Catal B 2023;328:122486.
38. Khan H, Lone IH, Lofland SE, Ramanujachary KV, Ahmad T. Exploiting multiferroicity of TbFeO3 nanoparticles for hydrogen generation through photo/electro/photoelectro-catalytic water splitting. Int J Hydrogen Energy 2023;48:5493-505.
39. Han Q, Han Z, Wang Y, et al. Enhanced photocatalytic hydrogen evolution by piezoelectric effects based on MoSe2/Se-decorated CdS nanowire edge-on heterostructure. J Colloid Interface Sci 2023;630:460-72.
40. Kai J, Saito R, Terabaru K, Li H, Nakajima H, Ito K. Effect of temperature on the performance of polymer electrolyte membrane water electrolysis: numerical analysis of electrolysis voltage considering gas/liquid two-phase flow. J Electrochem Soc 2019;166:F246.
41. Barco-burgos J, Eicker U, Saldaña-robles N, Saldaña-robles A, Alcántar-camarena V. Thermal characterization of an alkaline electrolysis cell for hydrogen production at atmospheric pressure. Fuel 2020;276:117910.
42. Tang S, Xing X, Yu W, et al. Synergizing photo-thermal H2 and photovoltaics into a concentrated sunlight use. iScience 2020;23:101012.
43. Mateo D, Cerrillo JL, Durini S, Gascon J. Fundamentals and applications of photo-thermal catalysis. Chem Soc Rev 2021;50:2173-210.
44. Yu F, Wang C, Li Y, et al. Enhanced solar photothermal catalysis over solution plasma activated TiO2. Adv Sci 2020;7:2000204.
45. Ghoussoub M, Xia M, Duchesne PN, Segal D, Ozin G. Principles of photothermal gas-phase heterogeneous CO2 catalysis. Energy Environ Sci 2019;12:1122-42.
46. Xiao JD, Jiang HL. Metal-organic frameworks for photocatalysis and photothermal catalysis. Acc Chem Res 2019;52:356-66.
47. Niu F, Wang D, Li F, Liu Y, Shen S, Meyer TJ. Hybrid photoelectrochemical water splitting systems: from interface design to system assembly. Adv Energy Mater 2020;10:1900399.
48. Tayebi M, Lee B. Recent advances in BiVO4 semiconductor materials for hydrogen production using photoelectrochemical water splitting. Renew Sustain Energy Rev 2019;111:332-43.
49. Maeda K, Domen K. Photocatalytic water splitting: recent progress and future challenges. J Phys Chem Lett 2010;1:2655-61.
50. Sang Y, Zhao Z, Zhao M, Hao P, Leng Y, Liu H. From UV to near-infrared, WS2 nanosheet: a novel photocatalyst for full solar light spectrum photodegradation. Adv Mater 2015;27:363-9.
51. Zhang J, Chen H, Duan X, Sun H, Wang S. Photothermal catalysis: from fundamentals to practical applications. Mater Today 2023;68:234-53.
52. Wang X, Ma S, Liu B, Wang S, Huang W. Imperfect makes perfect: defect engineering of photoelectrodes towards efficient photoelectrochemical water splitting. Chem Commun 2023;59:10044-66.
53. Wang Y, Zhang J, Balogun M, Tong Y, Huang Y. Oxygen vacancy-based metal oxides photoanodes in photoelectrochemical water splitting. Mater Today Sustain 2022;18:100118.
54. Zhao L, Liu Y, Xing R, Yan X. Supramolecular photothermal effects: a promising mechanism for efficient thermal conversion. Angew Chem Int Ed 2020;59:3793-801.
55. Seh ZW, Kibsgaard J, Dickens CF, Chorkendorff I, Nørskov JK, Jaramillo TF. Combining theory and experiment in electrocatalysis: insights into materials design. Science 2017;355:eaad4998.
56. Kim JH, Lee JS. Elaborately modified BiVO4 photoanodes for solar water splitting. Adv Mater 2019;31:e1806938.
57. Li Z, Luo W, Zhang M, Feng J, Zou Z. Photoelectrochemical cells for solar hydrogen production: current state of promising photoelectrodes, methods to improve their properties, and outlook. Energy Environ Sci 2013;6:347-70.
58. Stepanov IA. The heats of chemical reactions: the Van't-Hoff equation and calorimetry. Z Phy Chem 2005;219:1089-97.
59. Kweon KE, Hwang GS. Structural phase-dependent hole localization and transport in bismuth vanadate. Phys Rev B 2013;87:205202.
60. Tan HL, Amal R, Ng YH. Alternative strategies in improving the photocatalytic and photoelectrochemical activities of visible light-driven BiVO4: a review. J Mater Chem A 2017;5:16498-521.
61. Matthies M, Beulke S. Considerations of temperature in the context of the persistence classification in the EU. Environ Sci Eur 2017;29:15.
62. Pendlebury SR, Barroso M, Cowan AJ, et al. Dynamics of photogenerated holes in nanocrystalline α-Fe2O3 electrodes for water oxidation probed by transient absorption spectroscopy. Chem Commun 2011;47:716-8.
63. Cowan AJ, Barnett CJ, Pendlebury SR, et al. Activation energies for the rate-limiting step in water photooxidation by nanostructured α-Fe2O3 and TiO2. J Am Chem Soc 2011;133:10134-40.
64. Sleutels TH, Darus L, Hamelers HV, Buisman CJ. Effect of operational parameters on coulombic efficiency in bioelectrochemical systems. Bioresour Technol 2011;102:11172-6.
65. Harmon M, Gamba IM, Ren K. Numerical algorithms based on Galerkin methods for the modeling of reactive interfaces in photoelectrochemical (PEC) solar cells. J Comput Phys 2016;327:140-67.
66. Kreider ME, Gallo A, Back S, et al. Precious metal-free nickel nitride catalyst for the oxygen reduction reaction. ACS Appl Mater Interfaces 2019;11:26863-71.
67. Liu C, Wang Z, Zhang T, Zhang Y, Su J. Photo/thermal dual-activation improves the photocurrent of bismuth vanadate for pec water splitting. ChemElectroChem 2022;9:e202200646.
68. Ye X. Elevated temperature photo-electrochemical water splitting. 2016. Available from: http://purl.stanford.edu/vy785kk1866 [Last accessed on 30 Nov 2023].
69. Isik M, Delice S, Gasanly N. Temperature-dependent optical properties of TiO2 nanoparticles: a study of band gap evolution. Opt Quant Electron 2023;55:905.
70. Landman A, Dotan H, Shter GE, et al. Photoelectrochemical water splitting in separate oxygen and hydrogen cells. Nat Mater 2017;6:646-51.
71. Zhao H, Tian F, Wang R, Chen R. A review on bismuth-related nanomaterials for photocatalysis. Rev Adv Sci Eng 2014;3:3-27.
73. Leszczynski M, Suski T, Teisseyre H, et al. Thermal expansion of gallium nitride. J Appl Phys 1994;76:4909-11.
74. Hsu K, Wang C, Liu C. The growth of GaN nanorods with different temperature by molecular beam epitaxy. J Electrochem Soc 2010;157:K109.
75. Ye CH, Fang XS, Wang M, Zhang LD. Temperature-dependent photoluminescence from elemental sulfur species on ZnS nanobelts. J Appl Phys 2006;99:063504.
76. Pejova B, Abay B, Bineva I. Temperature dependence of the band-gap energy and sub-band-gap absorption tails in strongly quantized ZnSe nanocrystals deposited as thin films. J Phys Chem C 2010;114:15280-91.
77. Lee MG, Kim DH, Sohn W, et al. Conformally coated BiVO4 nanodots on porosity-controlled WO3 nanorods as highly efficient type II heterojunction photoanodes for water oxidation. Nano Energy 2016;28:250-60.
78. Tang R, Yin R, Zhou S, et al. Layered MoS2 coupled MOFs-derived dual-phase TiO2 for enhanced photoelectrochemical performance. J Mater Chem A 2017;5:4962-71.
79. Lee SA, Lee TH, Kim C, et al. Tailored NiOx/Ni cocatalysts on silicon for highly efficient water splitting photoanodes via pulsed electrodeposition. ACS Catal 2018;8:7261-9.
80. Chen D, Li X, Huang J, Chen Y, Liu Z, Huang Y. Boosting charge transfer of Fe doping BiVO4/CoOx for photoelectrochemical water splitting. ACS Appl Energy Mater 2023;6:8495-502.
81. Pejova B, Abay B. Nanostructured CdSe films in low size-quantization regime: temperature dependence of the band gap energy and sub-band gap absorption tails. J Phys Chem C 2011;115:23241-55.
82. Zhang L, Ye X, Boloor M, Poletayev A, Melosh NA, Chueh WC. Significantly enhanced photocurrent for water oxidation in monolithic Mo: BiVO4/SnO2/Si by thermally increasing the minority carrier diffusion length. Energy Environ Sci 2016;9:2044-52.
83. Tang S, Qiu W, Xu X, et al. Harvesting of infrared part of sunlight to enhance polaron transport and solar water splitting. Adv Funct Materials 2022;32:2110284.
84. Zhou C, Zhang L, Tong X, Liu M. Temperature effect on photoelectrochemical water splitting: a model study based on BiVO4 photoanodes. ACS Appl Mater Interfaces 2021;13:61227-36.
85. Wang Z, Yang C, Lin T, et al. Visible-light photocatalytic, solar thermal and photoelectrochemical properties of aluminium-reduced black titania. Energy Environ Sci 2013;6:3007-14.
86. Dias P, Lopes T, Andrade L, Mendes A. Temperature effect on water splitting using a Si-doped hematite photoanode. J Power Sources 2014;272:567-80.
87. Ye X, Yang J, Boloor M, Melosh NA, Chueh WC. Thermally-enhanced minority carrier collection in hematite during photoelectrochemical water and sulfite oxidation. J Mater Chem A 2015;3:10801-10.
88. He B, Jia S, Zhao M, et al. General and robust photothermal-heating-enabled high-efficiency photoelectrochemical water splitting. Adv Mater 2021;33:2004406.
89. Zhang Q, Ning X, Fan Y, et al. Insight into interface charge regulation through the change of the electrolyte temperature toward enhancing photoelectrochemical water oxidation. J Colloid Interface Sci 2021;588:31-9.
90. He B, Zhao F, Yi P, et al. Spinel-oxide-integrated BiVO4 photoanodes with photothermal effect for efficient solar water oxidation. ACS Appl Mater Interfaces 2021;13:48901-12.
91. Huang J, Hu X, Wang J, et al. Unraveling photothermal-enhanced bulk charge transport and surface oxygen reactions in TiO2 photoanodes for highly efficient photoelectrochemical water oxidation. Chem Eng J 2023;462:142246.
92. Hu X, Huang J, Cao Y, et al. Photothermal-boosted polaron transport in Fe2O3 photoanodes for efficient photoelectrochemical water splitting. Carbon Energy 2023;5:e369.
93. Lu X, Ye K, Zhang S, et al. Amorphous type FeOOH modified defective BiVO4 photoanodes for photoelectrochemical water oxidation. Chem Eng J 2022;428:131027.
94. Zhang Y, Huang Y, Zhu SS, et al. Covalent S-O bonding enables enhanced photoelectrochemical performance of Cu2S/Fe2O3 heterojunction for water splitting. Small 2021;17:2100320.
95. Xue F, Wu H, Liu Y, et al. CuS nanosheet-induced local hot spots on g-C3N4 boost photocatalytic hydrogen evolution. Int J Hydrog Energy 2023;48:6346-57.
96. Zhao F, Sheng H, Sun Q, et al. Harvesting the infrared part of solar light to promote charge transfer in Bi2S3/WO3 photoanode for enhanced photoelectrochemical water splitting. J Colloid Interface Sci 2022;621:267-74.
97. Deng C, Peng L, Ling X, et al. Construction of S-scheme Zn0.2Cd0.8S/biochar aerogel architectures for boosting photocatalytic hydrogen production under sunlight irradiation. J Clean Prod 2023;414:137616.
98. Tai Z, Sun G, Zhang X, et al. Embedding laser-generated CdTe nanocrystals into ultrathin ZnIn2S4 nanosheets with sulfur vacancies for boosted photocatalytic H2 evolution. J Mater Sci Technol 2023;166:113-22.
99. Wang K, Liu J, Tao Y, Benetti D, Rosei F, Sun X. Temperature-dependence photoelectrochemical hydrogen generation based on alloyed quantum dots. J Phys Chem C 2022;126:174-82.
100. Li L, Shi H, Yu H, et al. Ultrathin MoSe2 nanosheet anchored CdS-ZnO functional paper chip as a highly efficient tandem Z-scheme heterojunction photoanode for scalable photoelectrochemical water splitting. Appl Catal B 2021;292:120184.
101. Guo MJ, Zhao TY, Xing ZP, et al. Hollow Octahedral Cu2-xS/CdS/Bi2S3 p-n-p type tandem heterojunctions for efficient photothermal effect and robust visible-light-driven photocatalytic performance. Acs Appl Mater Interfaces 2020;12:40328-38.
102. Zhong W, Wang C, Zhao H, et al. Synergistic effect of photo-thermal catalytic glycerol reforming hydrogen production over 2D Au/TiO2 nanoflakes. Chem Eng J 2022;446:137063.
103. Valenti M, Jonsson MP, Biskos G, Schmidt-ott A, Smith WA. Plasmonic nanoparticle-semiconductor composites for efficient solar water splitting. J Mater Chem A 2016;4:17891-912.
104. Cushing SK, Li J, Bright J, et al. Controlling plasmon-induced resonance energy transfer and hot electron injection processes in metal@TiO2 core-shell nanoparticles. J Phys Chem C 2015;119:16239-44.
105. Lee S, Sun Y, Cao Y, Kang SH. Plasmonic nanostructure-based bioimaging and detection techniques at the single-cell level. Trends Analyt Chem 2019;117:58-68.
106. Zhang Z, Zhang L, Hedhili MN, Zhang H, Wang P. Plasmonic gold nanocrystals coupled with photonic crystal seamlessly on TiO2 nanotube photoelectrodes for efficient visible light photoelectrochemical water splitting. Nano Lett 2013;13:14-20.
107. Liu ZW, Hou WB, Pavaskar P, Aykol M, Cronin SB. Plasmon resonant enhancement of photocatalytic water splitting under visible illumination. Nano Lett 2011;11:1111-16.
108. Tang T, Li M, Liang Z, et al. Promoting plasmonic hot hole extraction and photothermal effect for the oxygen evolution reactions. Chemistry 2023;29:e202300225.
109. Agarwal D, Aspetti CO, Cargnello M, et al. Engineering localized surface plasmon interactions in gold by silicon nanowire for enhanced heating and photocatalysis. Nano Lett 2017;17:1839-45.
110. Huang Y, Long B, Tang M, et al. Bifunctional catalytic material: an ultrastable and high-performance surface defect CeO2 nanosheets for formaldehyde thermal oxidation and photocatalytic oxidation. Appl Catal B 2016;181:779-87.
111. Subramanyam P, Meena B, Sinha GN, Deepa M, Subrahmanyam C. Decoration of plasmonic Cu nanoparticles on WO3/Bi2S3 QDs heterojunction for enhanced photoelectrochemical water splitting. Int J Hydrog Energy 2020;45:7706-15.
112. Saeed S, Siddique H, Dai R, et al. Enhanced PEC water splitting performance of silver nanoparticle-coated CdS nanowire photoanodes: the role of silver deposition. J Phys Chem C 2021;45:7542-51.
113. Gelija D, Loka C, Goddati M, Bak N, Lee J, Kim MD. Integration of Ag plasmonic metal and WO3/InGaN heterostructure for photoelectrochemical water splitting. Acs Appl Mater Interfaces 2023;15:34883-94.
114. Song R, Liu M, Luo B, Geng J, Jing D. Plasmon-induced photothermal effect of sub-10-nm Cu nanoparticles enables boosted full-spectrum solar H2 production. AIChE J 2020;66:e17008.
115. Li J, Hatami M, Huang Y, Luo B, Jing D, Ma L. Efficient photothermal catalytic hydrogen production via plasma-induced photothermal effect of Cu/TiO2 nanoparticles. Int J Hydrog Energy 2023; 48:6336-45.
116. Subramanyam P, Meena B, Suryakala D, Deepa M, Subrahmanyam C. Plasmonic nanometal decorated photoanodes for efficient photoelectrochemical water splitting. Catal Today 2021;379:1-6.
117. Wu J, Xu X, Guo X, Xie W, Pan L, Chen Y. Polypyrrole modification on BiVO4 for photothermal-assisted photoelectrochemical water oxidation. J Chem Phys 2023;158:091102.
118. Zhao M, Chen T, He B, et al. Photothermal effect-enhanced photoelectrochemical water splitting of a BiVO4 photoanode modified with dual-functional polyaniline. J Mater Chem A 2020;8:15976-83.
119. Xu W, Meng L, Tian W, Li S, Cao F, Li L. Polypyrrole serving as multifunctional surface modifier for photoanode enables efficient photoelectrochemical water oxidation. Small 2022;18:2105240.
120. Hu C, Dai L. Carbon-based metal-free catalysts for electrocatalysis beyond the ORR. Angew Chem Int Ed 2016;55:11736-58.
121. Li T, Guo Z, Ruan M, Zou Y, Liu Z. Doping regulating spontaneous polarization and pyroelectric effects to synergistically promote the water splitting efficiency of niobate (KxNa1-xNbO3) pyro-photo-electrical coupling system. Appl Surf Sci 2022;592:153255.
122. Moon HK, Lee SH, Choi HC. In vivo near-infrared mediated tumor destruction by photothermal effect of carbon nanotubes. ACS Nano 2009;3:3707-13.
123. Wang Y, Chen D, Zhang J, et al. Charge relays via dual carbon-actions on nanostructured BiVO4 for high performance photoelectrochemical water splitting. Adv Funct Mater 2022;32:2112738.
124. Hu XQ, Huang J, Zhao FF, et al. Photothermal effect of carbon quantum dots enhanced photoelectrochemical water splitting of hematite photoanodes. J Mater Chem A 2020;8:14915-20.
125. Cai J, Tang X, Liu C, et al. Bifunctional photothermal effect to promote band bending and water oxidation kinetics for improving photoelectrochemical water splitting. Sol Energy Mater Sol Cells 2023;257:112360.
126. Noureen L, Xie Z, Hussain M, et al. BiVO4 and reduced graphene oxide composite hydrogels for solar-driven steam generation and decontamination of polluted water. Sol Energy Mater Sol Cells 2021;222:110952.
127. Wu Y, Sun Y, Fu W, et al. Graphene-based modulation on the growth of urchin-like Na2Ti3O7 microspheres for photothermally enhanced H2 generation from ammonia borane. ACS Appl Nano Mater 2020;3:2713-22.
128. Fang J, Sun F, Kheradmand A, et al. Solar thermo-photo catalytic hydrogen production from water with non-metal carbon nitrides. Fuel 2023;353:129277.
129. Wang M, Wan Z, Li Z, et al. Full spectrum solar hydrogen production by tandems of perovskite solar cells and photothermal enhanced electrocatalysts. Chem Eng J 2023;460:141702.
130. Qi J, Zhang W, Cao R. A new strategy for solar-to-hydrogen energy conversion: photothermal-promoted electrocatalytic water splitting. ChemElectroChem 2019;6:2762-5.
131. Xie X, Wang R, Ma Y, et al. Photothermal-effect-enhanced photoelectrochemical water splitting in MXene-nanosheet-modified ZnO nanorod arrays. ACS Appl Nano Mater 2022;5:11150-9.
132. Zhang Y, Song X, Xue S, Liang Y, Jiang H. Fabrication of hierarchically structured S-doped NiFe hydroxide/oxide electrodes for solar-assisted oxygen evolution reaction in seawater splitting. Appl Catal A 2023;649:118965.
133. Zhang Y, Hu L, Zhang Y, Wang X, Wang H. Snowflake-Like Cu2S/MoS2/Pt heterostructure with near infrared photothermal-enhanced electrocatalytic and photoelectrocatalytic hydrogen production. Appl Catal B 2022;315:121540.
134. Cao A, Sang L, Yu Z, et al. Investigation of the local photothermal effects by fabricating a CQDs/Au/TiO2 photoelectrode in a PEC water splitting system. Catal Sci Technol 2022;12:1859-68.
135. Zhao Y, Sang L, Wang C. Thermoplasmonics effect of Au-rGO/TiO2 photoelectrode in solar-hydrogen conversion. Sol Energy Mater Sol Cells 2023;255:112306.
136. Landman A, Halabi R, Dias P, et al. Decoupled photoelectrochemical water splitting system for centralized hydrogen production. Joule 2020;4:448-71.
137. Pareek A, Dom R, Borse PH. Fabrication of large area nanorod like structured CdS photoanode for solar H2 generation using spray pyrolysis technique. Int J Hydrog Energy 2013;38:36-44.
138. Vilanova A, Dias P, Azevedo J, et al. Solar water splitting under natural concentrated sunlight using a 200 cm2 photoelectrochemical-photovoltaic device. J Power Sources 2020;454:227890.
139. Song R, Luo B, Geng J, Song D, Jing D. Photothermocatalytic hydrogen evolution over Ni2P/TiO2 for full-spectrum solar energy conversion. Ind Eng Chem Res 2018;57:7846-54.
140. Hu S, Geng J, Jing D. Photothermal effect promoting photocatalytic process in hydrogen evolution over graphene-based nanocomposite. Top Catal 2021.
141. Gao M, Connor PKN, Ho GW. Plasmonic photothermic directed broadband sunlight harnessing for seawater catalysis and desalination. Energy Environ Sci 2016;9:3151-60.
142. Hu S, Shi J, Luo B, Ai C, Jing D. Significantly enhanced photothermal catalytic hydrogen evolution over Cu2O-rGO/TiO2 composite with full spectrum solar light. J Colloid Interface Sci 2022;608:2058-65.
143. Zhang L, Zhang X, Mo H, et al. Synergistic modulation between non-thermal and thermal effects in photothermal catalysis based on modified In2O3. ACS Appl Mater Interfaces 2023;15:39304-18.
144. Ding L, Li K, Li J, et al. Integrated coupling utilization of the solar full spectrum for promoting water splitting activity over a CIZS semiconductor. ACS Nano 2023;17:11616-25.
145. Han H, Meng X. Hydrothermal preparation of C3N4 on carbonized wood for photothermal-photocatalytic water splitting to efficiently evolve hydrogen. J Colloid Interface Sci 2023;650:846-56.
146. Li J, Ding L, Su Z, et al. Non-lignin constructing the gas-solid interface for enhancing the photothermal catalytic water vapor splitting. Adv Mater 2023;35:e2305535.
147. Wu Z, Li C, Li Z, et al. Niobium and titanium carbides (MXenes) as Superior photothermal supports for CO2 photocatalysis. ACS Nano 2021;15:5696-705.
148. Zhang J, Li M, Tan X, et al. Confined FeNi alloy nanoparticles in carbon nanotubes for photothermal oxidative dehydrogenation of ethane by carbon dioxide. Appl Catal B 2023;339:123166.
149. Zhang J, Xie K, Jiang Y, et al. Photoinducing different mechanisms on a Co-Ni bimetallic alloy in catalytic dry reforming of methane. ACS Catal 2023;13:10855-65.
150. Huang Y, Hu H, Wang S, Balogun M, Ji H, Tong Y. Low concentration nitric acid facilitate rapid electron-hole separation in vacancy-rich bismuth oxyiodide for photo-thermo-synergistic oxidation of formaldehyde. Appl Catal B 2017;218:700-8.
151. Huang Y, Lu Y, Lin Y, et al. Cerium-based hybrid nanorods for synergetic photo-thermocatalytic degradation of organic pollutants. J Mater Chem A 2018;6:24740-7.
152. Xiong R, Tang C, Li K, et al. Plasmon photothermal-promoted solar photocatalytic hydrogen production over a CoCr2O4/g-C3N4 heterojunction. J Mater Chem A 2022;10:22819-33.
153. Lin Z, Gao Q, Diao P. Promoting the electrocatalytic oxygen evolution reaction on NiCo2O4 with infrared-thermal effect: a strategy to utilize the infrared solar energy to reduce activation energy during water splitting. J Colloid Interface Sci 2023;638:54-62.
154. Dong B, Li F, Feng S. A visible-IR responsive BiVO4/TiO2 photoanode with multi-effect point defects for photothermal enhancement of photoelectrochemical water splitting. Chem Commun 2022;58:1621-4.
155. Zhang H, Lu Y, Han W, Zhu J, Zhang Y, Huang W. Solar energy conversion and utilization: towards the emerging photo-electrochemical devices based on perovskite photovoltaics. Chem Eng J 2020;393:124766.
156. Kim S, Piao G, Han DS, Shon HK, Park H. Solar desalination coupled with water remediation and molecular hydrogen production: a novel solar water-energy nexus. Energy Environ Sci 2018;11:344-53.
157. Cai H, Li L, Ni H, Xiao G, Yue Z, Jiang F. GeSe-based photovoltaic thin film photoelectrode for natural seawater desalination. Sep Purif Technol 2023;318:124034.
158. Kim YJ, Hong H, Yun J, Kim SI, Jung HY, Ryu W. Photosynthetic nanomaterial hybrids for bioelectricity and renewable energy systems. Adv Mater 2021;33:e2005919.
159. Zhou Y, Ma Y, Wang X, et al. Leaf-structure-inspired through-hole electrode with boosted mass transfer and photothermal effect for oxygen evolution reactions. Adv Funct Mater 2023;33:2304296.
Cite This Article
Export citation file: BibTeX | RIS
OAE Style
Zhang J, Tang T, Yang R, Wang G, Ye KH, Shi J. Photothermal effect and application of photothermal materials in photocatalysis and photoelectric catalysis. Microstructures 2024;4:2024008. http://dx.doi.org/10.20517/microstructures.2023.51
AMA Style
Zhang J, Tang T, Yang R, Wang G, Ye KH, Shi J. Photothermal effect and application of photothermal materials in photocatalysis and photoelectric catalysis. Microstructures. 2024; 4(1): 2024008. http://dx.doi.org/10.20517/microstructures.2023.51
Chicago/Turabian Style
Zhang, Jingnan, Tongxin Tang, Rongge Yang, Guilin Wang, Kai-Hang Ye, Jianxin Shi. 2024. "Photothermal effect and application of photothermal materials in photocatalysis and photoelectric catalysis" Microstructures. 4, no.1: 2024008. http://dx.doi.org/10.20517/microstructures.2023.51
ACS Style
Zhang, J.; Tang T.; Yang R.; Wang G.; Ye K.H.; Shi J. Photothermal effect and application of photothermal materials in photocatalysis and photoelectric catalysis. Microstructures. 2024, 4, 2024008. http://dx.doi.org/10.20517/microstructures.2023.51
About This Article
Special Issue
Copyright
Data & Comments
Data
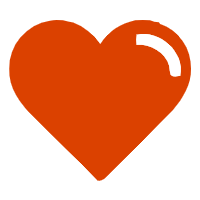

Comments
Comments must be written in English. Spam, offensive content, impersonation, and private information will not be permitted. If any comment is reported and identified as inappropriate content by OAE staff, the comment will be removed without notice. If you have any queries or need any help, please contact us at support@oaepublish.com.