Research progress of amorphous catalysts in the field of electrocatalysis
Abstract
Amorphous materials feature unique structures and physicochemical properties, resulting in their synthesis and applications becoming a dynamic and fascinating new research direction. The high specific surface area, abundant active sites, and good electron transport properties endow amorphous materials with excellent electrocatalytic properties, thus appealing to increasing attention. Based on this, the summary of the current research status of amorphous catalysts in the field of electrocatalysis is urgent and important. In this review, the research progress of amorphous catalysts in electrocatalysis is systematically introduced, focusing on the classification, synthesis methods, modification strategies, characterizations, and electrocatalytic application (including hydrogen evolution reaction, oxygen evolution reaction, oxygen reduction reaction, carbon dioxide reduction reaction, and nitrogen reduction reaction). Finally, this review proposes the prospects and challenges for the future development of high-active and high-selectivity amorphous electrocatalysts.
Keywords
INTRODUCTION
To realize the goals of carbon neutrality and carbon peaking, effectively improving the complex international situation, stabilizing the fossil fuel transportation conditions, and decreasing traditional energy use are at the heart of the matter[1-9]. A feasible strategy is to develop clean and sustainable energy systems via electrocatalysis that can both produce and store energy[10-15]. Compared to traditional energy conversion and storage, electrocatalysis features the merits of high efficiency[16], low carbon emissions[17], and controllability. Importantly, electrocatalysis can utilize intermittent solar and wind energy to drive sustainable energy conversion and storage[18-20], extremely mitigating energy and ecological crises. The sustainable energy from different electrocatalysis processes relies on various reaction mechanisms[21-23], while their realization and ultimate commercialization must depend on the development of high-performance electrocatalysts. The design of highly active stability electrocatalysts needs to consider the following three factors: (1) Intrinsic activity[24]; (2) electron transfer capacity[25]; and (3) diffusion ability of the substance. As the widely recognized high-quality electrocatalysts, the large-scale applications of noble metals (Pt, Au, Ru, Ir, etc.)-based materials are severely limited by low reserve and high price[26,27]. Thus, designing high-active and low-cost innovative electrocatalysts is crucial for the development of sustainable energy and ecological systems.
Recently, amorphous catalysts have exhibited unique structural and chemical properties, becoming promising electrocatalytic candidates and receiving a growing interest and potential in oxygen evolution reaction (OER), hydrogen evolution reaction (HER), oxygen reduction reaction (ORR), carbon dioxide reduction reaction (CO2RR), nitrogen reduction reaction (NRR), and so on[28-32]. The tunability in composition, morphology, active sites, and surface properties provides an attractive platform to improve catalytic activity, stability, and selectivity. As illustrated in Figure 1A, the factors in amorphous catalysts that facilitate electrocatalytic performances are superior to those of crystalline catalysts. In detail, amorphous materials feature short-range order but long-range disorder, resulting in high-density defects and high surface area[33]. The increased surface area endows amorphous catalysts with more active sites for catalytic reactions and exhibits enhanced catalytic activity. Secondly, the tunable composition makes it easy to incorporate heteroatoms in amorphous catalysts, thus significantly enhancing catalytic properties[34]. Thirdly, the disordered atomic structure of amorphous catalysts promotes efficient charge transfer[35], reactant diffusion, and adsorption-desorption in reaction processes, thus improving catalytic activity. Meanwhile, the presence of defects and dislocations in the amorphous structure can act as active sites, leading to enhanced intrinsic activity of catalysts[36,37]. Fourthly, the absence of grain boundaries and lattice defects restrains amorphous structural degradation and corrosion, delivering long operation stability and catalytic activity at harsh reaction conditions with almost no attenuation[38]. Fifthly, the synthesized methods of amorphous catalysts show diversity, such as sol-gel techniques[39], chemical vapor deposition[40], electrodeposition, etc.[41]. These strategies enable amorphous catalysts to tune specific surface morphology, composition, and porosity, which can then be applied to a variety of electrocatalytic reactions. Lastly, compared to crystalline catalysts, the less expensive and abundant precursors endow amorphous catalysts with low cost. The advantages result in the large-scale industrial applications of amorphous catalysts, making them economically viable and becoming a kind of sustainable candidate electrocatalysts.
Figure 1. (A) The main characteristic comparison of amorphous and crystalline catalysts. (B) Overview of amorphous catalysts in electrocatalysis.
Per the above analysis, amorphous catalysts exhibit huge application potential in electrocatalysis. In this review, we provide a systematic overview of the recent advances in amorphous catalysts in HER, OER, ORR, CO2RR, and NRR [Figure 1B]. We first introduced the classification of amorphous catalysts and secondly outlined general preparation methods for amorphous catalysts. Next, we summarized the experimental and theoretical characterizations of amorphous catalysts. We then elaborate on the modification strategies of amorphous catalysts, such as structural design, composition tuning, surface modification, interface engineering, etc. Moreover, we introduce the applications of amorphous catalysts in various electrocatalytic reactions. Finally, the current challenges and prospects of amorphous catalysts are discussed. We hope this review sheds light on the design of high-active amorphous electrocatalysts and stimulates their extensive applications.
CLASSIFICATION OF AMORPHOUS CATALYSTS
In catalytic reactions, crystalline electrocatalysts are commonly used. This is a class of highly crystalline catalytic materials with a regular lattice structure and well-defined crystal surface orientation, which usually exhibit high catalytic activity and selectivity and have good catalytic effects for some catalytic reactions. Their lattice structure and crystal plane orientation can provide good surface active sites, which is favorable for the catalytic reactions[42]. Common crystalline catalysts include spinel-type, chalcogenide-type, etc. An effective bifunctional catalyst for overall water splitting was established using a mesoporous NiFe-oxide nanocube (NiFe-NC) system based on NiFe Prussian blue analog metal-organic frameworks (MOFs)[43]. When water splitting was tested in an alkaline solution, the overpotentials of NiFe-NCs for HER and OER reached 197 and 271 mV, respectively, at a current density of 10 mAcm-2, indicating good catalytic performance. A novel lanthanide-based high-entropy chalcogenide (HEPO) electrocatalyst for oxygen precipitation reaction was reported by Nguyen et al.[44]. The optimized La(CrMnFeCo2Ni)O3 HEPO had an OER overpotential of 325 mV at a current density of 10 mA cm-2 and had excellent electrochemical stability after testing for 50 h. Single-phase nanoporous high-entropy alloy (HEA) np-AlNiCoFeX (X = Mo, Nb, Cr) catalysts with high-entropy (oxygen) hydroxide (HEO) overlayers prepared by combining precursor alloy design with chemical etching exhibited excellent OER activity by Qiu et al.[45]. AB2O4 (A = Zn2+;
However, with the continuous improvement of performance requirements for catalytic materials, people have discovered that there are certain limitations in achieving ideal catalytic effects solely by relying on crystal structure and crystal plane orientation[48] because, in catalytic reactions, the configuration of surface active sites, the surface defect structure of the catalyst, grain boundaries and intragranular defects may all affect the catalytic performance. To overcome these limitations, amorphous catalysts have become the focus of attention. Amorphous catalysts are catalytic materials with amorphous or amorphous structures, which are rich in defect structures, pore structures, and higher surface activity. This structural flexibility and tunability allows amorphous catalysts to be better adapted to different catalytic reactions and provide more active sites. For example, Altaf et al. first synthesized LaCoO3 with a chalcogenide structure, followed by an amorphization process via urea reduction and tailored modifications[49]. The results showed that the amorphous LaCoO3 (abbreviated as LCO-4) has a high electrochemical active area and exhibited excellent OER activity and stability superior to that of pristine LaCoO3, confirming that its unique disordered structure is one of the key factors in improving the catalytic performance.
In the history of amorphous structures in electrocatalysis, amorphous catalysts can be roughly divided into noble metal-based and transition metal-based amorphous catalysts. Taking cost and industrialization into consideration, recent research for amorphous catalysts is mostly focused on transition metal-based materials rather than noble metal-based materials.
Noble metal-based amorphous catalysts
Precious metal-based catalysts (such as Pt-based, Ir-based, Ru-based, etc.) are widely used as electrocatalysts; however, their high cost limits their large-scale applications. Optimizing their inherent activity, increasing active sites, and improving operational stability are urgent. Amorphization endows noble metal-based amorphous catalysts with unique surface and active site distribution, improving reaction efficiency and selectivity, thus receiving increasing attention in electrocatalysis. For example, excellent acidic OER performances were measured in amorphous IrOx (a-IrOx) thin film catalysts rather than crystalline IrOx thin films[50], whose overpotentials at 1 and 10 mA cm-2 were 190 and 220 mV, respectively. Moreover, Pd2RuOx materials[51] were synthesized by confining atomically thin Pd-PdO nanodomains in amorphous metallic ruthenium oxide (RuO2) NSs and then performing a reduction treatment [Figure 2A], which exhibited excellent electrocatalytic activity in electrolyzing water. The presence of Pd-PdO heterogeneous interfaces and numerous surface defects in materials facilitates active substance adsorption and thus promotes the electrocatalytic reaction [Figure 2B]. Na-modified defect-rich crystalline/amorphous rutile structure RuO2 (a/c-RuO2) catalysts delivered excellent OER catalytic stability [Figure 2C], benefiting from the abundant defects, grain boundaries, and accessibility of active sites[52].
Figure 2. (A) The synthesis of PdRuOx materials; (B) HRTEM image of Pd2RuOx-0.5 h. (Reproduced with permission[51]. Copyright 2023, WILEY-VCH). (C) Chronopotentiometry measurement results for a/c-RuO2 and commercial RuO2 at 10 mA cm-2 in 0.1 M HClO4. (Reproduced with permission[52]. Copyright 2021, WILEY-VCH). (D) High-magnification SEM images of Pt-a/c-NiCoHPi. (Reproduced with permission[53]. Copyright 2023, WILEY-VCH ). (E) Schematic illustration of the size-controllable synthesis of a-PdCu nanoparticles. (F) FE of different products; (G) Gibbs free energy diagram of the EOR on a-PdCu and c-PdCu model catalysts in the C1 path. (Reproduced with permission[54]. Copyright 2022, American Chemical Society).
Given the small reserves and expensive nature of precious metals, reducing their active loading without affecting the overall electrocatalytic efficiency is one of the cost-effective strategies. The Pt-a/c-NiCoHPi catalyst with crystalline/amorphous structure (Pt content is only 2.1%) exhibited excellent HER activity in alkaline solution, with an overpotential of only 19 mV at 10 mA cm-2 [Figure 2D][53]. Wang et al. synthesized amorphous PdCu nanorods with controllable size and morphology [Figure 2E] and used them for ethanol electrooxidation reaction[54]. The prepared amorphous PdCu nanorods delivered a high Faraday efficiency (FE) of 69.6% for C1 products, which was much higher than that of the corresponding crystalline catalysts and the commercial Pd/C [Figure 2F]. Density-functional-theory (DFT) calculations further revealed that the amorphous structure can significantly reduce the energy barrier for C-C bond breaking on the surface of the PdCu catalysts and promote the kinetic process of the ethanol oxidation reaction [Figure 2G]. Despite the remarkable progress of noble metal-based amorphous catalysts in the field of electrocatalysis, there are still challenges and issues, such as the high cost and stability of noble metal catalysts. Therefore, the development of amorphous catalyst materials with economic benefits and high reactivity is one of the hot spots for future research.
Transition metal-based amorphous catalysts
Thanks to the abundant earth reserves and cost-effectiveness, amorphous catalysts composed of non-noble transition metal elements have been widely studied in electrocatalysis. For instance, Jiang et al.[55] prepared self-supported FeNiCo-based amorphous catalysts with hierarchical micro/nanopore structures by dealloying amorphous/nanocrystalline precursors [Figure 3A]. The optimized electrocatalyst showed a HER overpotential of 134 mV and an OER overpotential of 206 mV at 10 mA cm-2 in alkaline solution. The main reason is that amorphous nanoporous frameworks can provide fast reaction kinetics, abundant active sites, and enhanced electrochemically active surface area. The synergistic effect of the structural and compositional advantages of the catalysts finally showed excellent catalytic activity.
Figure 3. (A) Schematic diagram of self-supported hierarchical porous amorphous FeNiCo-based bifunctional catalysts. (Reproduced with permission[55]. Copyright 2021, Elsevier). (B) TEM and (C) HRTEM image of MoSx@NCNFs-30 films. (Reproduced with permission[56]. Copyright 2016, Elsevier). (D) HRTEM diagram of amorphous CoOx nanoparticles (The insert shows its SAED patterns); (E-G) Free energy diagram for the water oxidation on Co3O4, CoOOH, and 1D-CoOx surfaces. (Reproduced with permission[57]. Copyright 2017, WILEY-VCH). (H) TEM image and SAED pattern (inset) of CoB@TiO2 nanosheets; (I) NH3 yields and FE at given potentials. (Reproduced with permission[59]. Copyright 2022, CCS).
Novel amorphous flower-like molybdenum sulfide@nitrogen doped carbon nanofiber (MoSx@NCNFs) thin films were synthesized and used to reduce water[56]. MoSx NSs immobilized on NCNFs in a unique flower-like structure were detected in the transmission electron microscope (TEM) image [Figure 3B], while the amorphous structure of MoSx was confirmed by selected area electron diffraction (SAED) (inset of Figure 3B) and Figure 3C. The MoSx@NCNFs exhibited excellent HER catalytic activity, with an overpotential of 137 mV at 10 mA cm-2. Amorphous cobalt oxide nanoparticles (ca. 2 nm) acted as water oxidation catalysts with a turnover frequency of up to 8.6 s-1 in the Ru(bpy)32+-Na2S2O8 system [Figure 3D], which is superior to that of the most active homogeneous cobalt-based molecules[57]. Meanwhile, theoretical calculations showed that the obtained structure promotes O-O bond coupling and improves the water oxidation activity compared with crystalline cobalt oxide [Figure 3E-G], further confirming the good activity of amorphous cobalt oxide nanoparticles. Pang et al. used a simple electrochemical dealloying method to prepare a self-supporting amorphous NiFeCoP catalyst with a nanoporous structure (np-NiFeCoP)[58]. The np-NiFeCoP catalyst exhibited good electrocatalytic activity from its unique structural characteristics, which can expose more active sites and exert synergistic effects between metal elements. In 1.0 M KOH alkaline solution, the overpotentials of HER and OER were 105 and 244 mV for np-NiFeCoP catalyst at 10 mA cm-2, respectively. Amorphous transition metal boride (TMB) nanomaterials featured numerous defects and dangling bonds, thus providing abundant active sites for the electrocatalytic nitrite reduction reaction (NO2-RR). CoB@TiO2/Ti plate (TP) was prepared by sputtering an amorphous cobalt boride nanofilm on the surface using titanium dioxide (TiO2) NS arrays as a substrate by Hu et al.
As one kind of emerging catalyst, transition metal-based amorphous catalysts present many unique properties and application potential. However, its industrialization and commercial applications still face several challenges, such as production cost, catalyst stability, and scale-up preparation. Therefore, future development requires further strengthening of the industrialization technology research and economic feasibility analysis.
Other types of amorphous catalysts
Recently, HEAs and high-entropy oxides (HEOs) composed of five or more elements have become a research hotspot in the field of catalysts due to their tunable electronic structure and wide range of component selection[60]. Among them, the design of multi-component high-entropy catalysts helps to improve the catalytic activity and stability of the catalysts. In addition, amorphous high-entropy catalysts have disordered local structures, which can provide more reaction active sites and exhibit superior catalytic performance. For example, Wang et al. prepared nanostructured amorphous Fe29Co27Ni23Si9B12 HEA strips and used them as electrocatalysts for OER [Figure 4A][61]. Thanks to their highly disordered configuration, they exhibited excellent catalytic activity in electrocatalytic activity tests, with an overpotential of only
Figure 4. (A) XRD patterns of various samples. (B) LSV polarization curves of different catalyst electrodes for the OER in 1.0 M KOH. (Reproduced with permission[61]. Copyright 2022, Springer). (C) Schematic diagram of HEO-Origin preparation. (D) TEM image and (E) SAED patterns of HEO-Origin. (Reproduced with permission[63]. Copyright 2023, Elsevier). (F) Schematic diagram of the synthesis of La2O3@NP-NS. (G) SAED pattern of
Rare earth elements have more unpaired electrons and irregular electron arrangement, which makes rare earth catalysts have high catalytic activity. Rare earth amorphous catalysts are an attractive class of catalysts with abundant active sites and defects in their structures, which provide more reaction sites and further enhance their catalytic activity. Yan et al. reported rare earth metal oxide electrocatalysts composed of ultrathin amorphous La2O3 NSs hybridized with uniform La2O3 nanoparticles [Figure 4F][64]. As shown in Figure 4G, the SAED pattern of the NS surfaces showed broad and diffuse diffraction rings, indicating that La2O3@ NP-NS indeed has a large number of amorphous phases with small crystals. Among them, the
SYNTHESIS METHOD OF AMORPHOUS CATALYSTS
In the history of amorphous catalyst preparation, numerous synthesized strategies have been developed and can be broadly categorized into liquid, vapor, and solid phases.
Liquid phase method
The liquid phase method dissolves the desired atoms or molecules in a liquid solution and then forms amorphous catalysts; the synthesis process needs to control parameters such as temperature, concentration, pH, etc.[67,68]. The atoms or molecules dissolve in the solution and combine to form an amorphous catalyst via chemical reactions or precipitations. This method features the merits of simple operation, easily controlled reaction conditions[69], diverse catalyst morphology, etc., thus receiving extensive attention. The typical liquid phase method contains the sol-gel method, deposition method, hydrothermal method, etc.
(1) Sol-gel method: The metal alkoxides or metal salts in a solution undergo hydrolysis and condensation to form a sol, followed by gelation and drying to obtain a solid material. This method has the advantages of low-temperature processing, high degree homogeneity, and excellent controllable catalyst size and morphology. For example, Zhang et al. used a sol-gel method to prepare a Prussian blue analog (NiFe-PBA) precursor with a two-dimensional (2D) network and then further annealed it into a Fe3O4/NiCx composite (NiFe-PBA-gel-cal), as a bifunctional electrocatalyst for seawater splitting [Figure 5A][70]. It can be seen from the high-resolution TEM (HRTEM) image of the calcined composite material that NiCx contains both amorphous and crystalline phases [Figure 5B], which is beneficial to the catalytic activity in electrochemical tests. Furthermore, the amorphous Cr8Co1-300 catalyst from the sol-gel method was reported[71], which exhibits a high specific surface area.
Figure 5. (A) Schematic diagram of the synthesis of NiFe-PBA-gel-cal. (B) HRTEM image of NiFe-PBA-gel-cal. (Reproduced with permission[70]. Copyright 2022, Wiley-VCH). (C) Schematic illustration of CoFe-H structure and its application in electrochemical and photo-electrochemical water oxidation as an OER electrocatalyst. (D) TEM, and (E) HRTEM images of CoFe-H nanosheets (the insert is corresponding FFT). (Reproduced with permission[72]. Copyright 2017, WILEY-VCH). (F) The fabrication illustration of Ru SAs-MoO3-x/NF. (G) XRD patterns of Ru SAs-MoO3-x/NF and Ru SAs-MoO3/NF. (Reproduced with permission[78]. Copyright 2023, WILEY-VCH).
(2) Deposition method: Depositing a metal precursor or its compound in a solution on a suitable carrier surface. This method includes the impregnation method, brush coating method, suspension deposition method, etc. Generally, amorphous metal alloys, carbon-based amorphous materials, amorphous nanocomposites, etc., can be prepared via the deposition method. For instance, amorphous cobalt iron hydroxide (CoFe-H) NSs were synthesized by electrodepositing CoFe-H NSs on graphite at 1.42 V
(3) Hydrothermal method: A direct method to prepare amorphous catalysts with no need for complex machines or working conditions[75,76]. Typically, the precursor only interacts with a solvent (often water) for a brief amount of time at a high temperature and high pressure to synthesize catalysts. The reaction conditions of high temperature and high pressure ensure the complete dispersion and dissolution of the solvent and precursor, benefiting the uniform reaction and the even distributed active sites. On the other hand, combining reaction temperature and time can tune the morphology, pore structure, and surface characteristics of amorphous catalysts, finally modifying catalytic performances. For example, the basic La3+ from the prepared bulk-phase LaNiO3 chalcogenide oxides would selectively dissolve in FeCl3 solution [reaction (1)][77]. With the consumption of H+, in the reaction process, part Fe3+ was hydrolyzed and deposited on the surface of the residual substrate [reaction (2)]. Noticeably, the La3+ in LaNiFe hydroxide stemmed from the residual substrate rather than the solution. The successive leaching of the large-sized La3+ ions induces the crystalline chalcogenide structure to gradually collapse and turn into an amorphous state, thus generating a novel amorphous Ni-Fe-based OER electrocatalyst. Moreover, the precursor grown on the surface of nickel foam (NF) by hydrothermal process was immersed in RuCl3 solution and annealed in air to prepare the amorphous molybdenum-based oxide stabilized single-atomic-site (Ru-SAs-MoO3-x/NF) catalysts [Figure 5F][78]. The X-ray diffraction (XRD) of the scraped powder from the Ru-SAs-MoO3-x/NF surface indicates almost no characteristic peaks [Figure 5G], suggesting its amorphous nature.
Vapor phase method
Amorphous catalysts from a vapor phase approach need to combine atoms or molecules in a specific ratio in an inert atmosphere before causing them to react on the catalyst surface. This method presents significant merits in terms of high purity[79], homogeneity[80], controllability, and specific surface area, facilitating the synthesis of amorphous catalysts with complex components. Typically, vapor phase methods can be classified as the physical vapor phase deposition (PVD) and chemical vapor phase (CVD).
Physical Vapor Deposition (PVD): This method refers to depositing thin metal or metal compound films onto a substrate by a physical process, such as sputtering, evaporation, pulsed laser deposition, etc.[81,82]. The attractiveness is the precise control of deposition conditions, allowing the formation of an amorphous film with unique structures and excellent catalytic properties. PVD shows outstanding advantages in terms of elaborately regulating the thickness and composition of amorphous films. For instance, a thin conformal amorphous IrOx (a-IrOx) coating was reported by Smith et al., which was produced at room temperature in an ambient atmosphere by the light-induced breakdown of a photoactive Ir(acac)3 precursor placed on a conducting substrate [Figure 6A][50]. In the XRD pattern, there are no diffraction peaks related to the iridium oxide crystal phase, confirming the amorphous nature of a-IrOx [Figure 6B]. Moreover, an oxygen-rich vacancy amorphous copper oxide nanofilm (m-CuOx) catalyst was prepared by a vacuum evaporation method[83], and its amorphous was indexed from the XRD spectrum.
Figure 6. (A) Schematic diagram of the preparation of amorphous IrOx. (B) X-ray diffraction patterns for a-IrOx and c-IrOx on FTO. (Reproduced with permission[50]. (Copyright 2014, American Chemical Society). (C) The general synthetic process of amorphous noble metal NSs. (D) TEM, and (E) Aberration-corrected HAADF-STEM image of amorphous Ir NSs (the inset in E shows the SAED pattern). (Reproduced with permission[87]. Copyright 2019, Nature).
(2) Chemical Vapor Deposition (CVD): This method means depositing metal atoms on a substrate surface by breaking down the gas-phase precursor compound in an inert gas atmosphere[84-86]. Generally, this method can be applied to amorphous metal oxides and carbon-based amorphous materials. For example, a variety of amorphous noble metal NSs less than 10 nm thickness was produced from direct annealing, such as amorphous Ir NSs, amorphous IrRu NSs, amorphous IrRhRu NSs, etc. [Figure 6C][87]. The annealed medium is a mixture of metal acetylpyruvates and alkali salts. As shown in Figure 6D and E, the amorphous feature of the prepared Ir NSs was confirmed by TEM and aberration-corrected High-Angle Annular Dark Field-Scanning Transmission Electron Microscopy (HAADF-STEM) characterizations.
Solid phase method
This method refers to preparing materials by using solid-state reactions[88]. In the solid-state reactions for forming amorphous catalysts, the spread and assembly of atoms or molecules can be realized by solid-phase diffusion or physically excited diffusion and aggregation. The solid-phase method, known for its advantages of simple preparation, high controllability, and homogeneity, facilitates the synthesis of amorphous catalysts[89]. Importantly, this method exhibits remarkable advantage in the synthesis of substances that have a high melting point or are unstable in solution. However, the solid-phase method faces several challenges of lower specific surface area, strong crystallinity, high influence from impurities, and strict preparation requirements. Conventionally, the solid phase method includes melt quenching, sputtering, and ball milling.
Melt-quenching method: The reactants first melt at high temperatures, followed by rapid quenching to room temperature or lower. The rapid cooling benefits to forming an amorphous structure rather than a long-range order structure[90]. Commonly, this method is conducted to synthesize amorphous metallic alloys by rapidly solidifying the metal-molten mixture. The prepared amorphous alloys own a high degree of compositional flexibility. For instance, amorphous Al80Pd10Cu5Ni5 strips with a thickness of 30~50 μm and a width of 2~4 mm [Figure 7A] were reported by Yang et al.[91]. In detail, an Al80Pd10Cu5Ni5 master alloy at Ar atmosphere was first prepared via arc melting. Subsequently, the master alloy in a quartz tube was remelted to a boiling state and sprayed onto a copper roll. Finally, the final amorphous nanoporous PdCuNi-S catalyst was formed by a two-step dealloying method. Moreover, amorphous Ni-Fe alloy strips with a thickness of 25 μm and a width of 2 mm were prepared by using the same synthesis method[92]. The amorphous catalysts from a melting-dumping strip technique feature changed structure and play a better role in the relevant electrocatalytic reactions. This method features the merits of high-speed solidification, uniformity, controllability, and high production efficiency technology, thus receiving wide applications.
Figure 7. (A) Synthetic procedure of nanoporous PdCuNi-S catalyst. (Reproduced with permission[91]. Copyright 2019, Elsevier). (B) Schematic diagram of depositing Ir25Ni33Ta42 MG film on Si substrates. (C) HRTEM image and its corresponding SAED pattern (inset) of the as-deposited Ir25Ni33Ta42 MG film. (Reproduced with permission[93]. Copyright 2019, Wiley-VCH). (D) Schematic illustration of the two-stage ball milling synthesis process for producing amorphous nanoparticles. (E-H) Nanobeam diffraction (NBD) patterns at the surface regions in Ni74.2Co5Nb12.5Y8.3 nanoparticles. (Reproduced with permission[95]. Copyright 2020, American Chemical Society).
Sputtering method: This method utilizes energetic particles to bombard the target material surface, resulting in the metal atoms dissociating from the target material and depositing onto the substrate. For example, an amorphous Ir25Ni33Ta42 nano-thick metallic glass (MG) film with low Ir content was grown on a Si substrate by using ion beam deposition (IBD) [Figure 7B][93]. The amorphous structure of the deposited Ir25Ni33Ta42 MG thin film was revealed by the HRTEM and related SAED characterizations [Figure 7C]. As a HER electrocatalyst, the obtained amorphous film exhibits high activity and stability in 0.5 M H2SO4 solution.
Ball milling method: A typical way to create amorphous catalysts and also known as mechanical alloying. This approach refers to conversing mechanical energy to the collision, friction, and shearing of solid feedstocks in a ball milling vessel; finally, the amorphous catalysts would be produced by mixing and reacting the feedstock powders at a tiny scale[94]. For instance, a two-stage ball milling process was used to prepare the amorphous Ni79.2-xCoxNb12.5Y8.3 (x = 0 and 5 at%) OER catalysts[95], and the precursor was the mixture of nickel (99.9 wt%, -100 mesh), cobalt (99.8 wt%, -100 mesh), niobium (99.99 wt%, -325 mesh), and yttrium (99.9 wt%, -40 mesh) metal element powders [Figure 7D]. The prepared amorphous catalysts deliver excellent structural stability [Figure 7E-H].
Based on the above description, we briefly summarize the preparation methods in Table 1.
Comparisons between three major preparation methods
Methods | Liquid phase method | Vapor phase method | Solid phase method |
Principle | Amorphous catalysts are formed by dissolving the desired atoms or molecules in a liquid solution to control reaction conditions | Materials are physically deposited onto a substrate in atomic or molecular form | Formation of amorphous catalyst materials under solid phase conditions by solid-state reactions |
Applicable conditions | Usually performed at relatively low temperatures and pressures | Suitable for deposition at high temperatures; certain vacuum conditions | Synthesize some high melting point metals |
Advantages | Simple operation; easily controlled reaction conditions | High homogeneity and controllability | Simple preparation; high controllability, etc. |
Disadvantages | Difficulty in structural control; low purity | Require high temperature conditions and high equipment costs | High requirements on raw material composition and mixing uniformity |
Categorizations | Sol-gel method: suitable for scenarios that require flexible control of ingredients and structure; Deposition method: suitable for preparing complex alloys or heterogeneous catalysts; Hydrothermal method: suitable for the preparation of hydroxides and hydroxide precursors to form particles or films of amorphous catalysts | PVD: typically used to prepare amorphous films with unique structures and excellent properties; CVD: preparation of amorphous catalysts with controlled gas phase composition and reaction conditions | Melt-quenching method: suitable for materials that require rapid cooling under high temperature conditions to form an amorphous structure; Sputtering method: suitable for controlled preparation of amorphous thin films under high vacuum conditions; Ball milling method: high-energy ball milling equipment and strict reaction environment control conditions are required |
Other novel methods
Based on the above analysis, the amorphous catalysts from most traditional methods face the issues of heterogeneity and uncontrollable texture. Hence, numerous novel preparation methods have been developed to obtain higher quality and stable amorphous catalysts. For example, the supersaturated precipitation method was applied to produce amorphous NiFeMo oxide with a mass of up to 515 g[96]. This method first mixes high-concentration metal chloride precursors (such as NiCl2·2H2O and FeCl3) in water. Then, 1 M Na2MoO4·2H2O aqueous solution was added to the mixture at ultrasonic conditions and reaction for 2 min; the samples were obtained via freeze-drying [Figure 8A]. Moreover, homogeneously mixing the high-temperature calcination-wet chemical synthesized highly crystalline LaCoO3 (LaCoO3-Pristine) nanopowders with urea in the mass ratio of 1:10, then heating at 450 °C for 2 h and cooling to room temperature can obtain reduced LaCoO3 powder (LaCoO3-Reduce) [Figure 8B][97]. The XRD pattern indicates the amorphous state of LaCoO3-Reduce after reducing by urea [Figure 8C]. The disordered structure of LaCoO3-Reduce in Figure 8D further demonstrates the amorphous character. Additionally, combining the merits of each preparation method is a new strategy to prepare amorphous catalysts. For example, amorphous TiO2 nanofibers (a-TiO2) were produced by combining the preparation methods of precursor solution[98], electrostatic spinning, and calcination [Figure 8E]. Moreover, combining hydrothermal with phosphating can synthesize MoO2/Ni3(PO4)2 self-supporting microcolumn electrocatalyst with amorphous MoOx phase at the NF surface (SA-MoO2/Ni3(PO4)2/NF)[99].
Figure 8. (A) The synthesis illustration of a-NiFeMo catalyst. (Reproduced with permission[96]. Copyright 2019, Wiley-VCH). (B) The preparation schematic of crystalline LaCoO3 and amorphous LaCoOx nanopowders. (C) XRD patterns of pristine and reduced LaCoO3 nanopowders. (D) TEM image of reduced LaCoO3 nanopowders. (Reproduced with permission[97]. Copyright 2022, American Chemical Society). (E) The schematic diagram for the formation of lotus-root-like a-TiO2 nanofibers and the atomic-scale deposition of Ga. (Reproduced with permission[98]. Copyright 2022, American Chemical Society).
CHARACTERIZATIONS OF AMORPHOUS CATALYSTS
The characterizations of amorphous catalysts can reveal the structure-activity relationship and then provide the experimental and theoretical basis for the design and applications of high-active stable amorphous electrocatalysts. Currently, the related characterizations can be divided into experiment and theory categories.
Experimental characterizations
A common characterization method of amorphous structures is XRD. The information on crystal structure and lattice parameters can be detected by the incident X-rays. However, the disordered crystal structure of amorphous induces steamed bun peaks occurring in XRD patterns[100,101], thus making it challenging to provide detailed structural information. Meanwhile, various amorphous catalysts always exhibit similar XRD patterns, resulting in the subtle differences between them indistinguishable via XRD. The micromorphology and fine structure of amorphous catalysts can be observed by TEM. The absence of clear lattice fringes in HRTEM means the disordered and irregular atomic arrangement in amorphous catalysts. The unit cell parameters or crystal face indexes cannot be deduced from HRTEM, which is different from crystalline catalysts. The diffraction ring or fuzzy core spots emerging in SAED can further verify the amorphous feature of catalysts. Similar to the crystalline catalysts, the band energy spectrum analysis from TEM can determine various element distributions in amorphous catalysts. Moreover, Zhan et al. reported that nanobeam electron diffraction (NBED) image of (NbTiZr)77.8Si22.2 (at.%) coating showed a broad diffraction ring indicating its amorphous nature [Figure 9A][102].
Figure 9. (A) The NBED pattern of (NbTiZr)77.8Si22.2 (at.%) coating. (Reproduced with permission[102]. Copyright 2022, Elsevier). (B) Cr K-edge XANES spectra and (C) Fourier-transforms of k3-weighted Cr K-edge EXAFS spectra in the R-space of H-CrOx/C-550, MIL-101, commercial Cr2O3, and Cr foil. (D) Wavelet transforms for the k3-weighted EXAFS signals of MIL-101, H-CrOx/C-550, and commercial Cr2O3. (Reproduced with permission[104]. Copyright 2022, American Chemical Society). (E) FTIR spectrum and (F) Raman spectrum of amorphous Fe-Mo-O/NF. (Reproduced with permission[105]. Copyright 2022, American Chemical Society). (G) N2 adsorption-desorption isotherms of NG and Cu/Fe-NG. Copyright 2022. (Reproduced with permission[106]. Copyright 2020, American Chemical Society).
As an important characterization, X-ray absorption near-edge structure (XANES) is derived from X-ray absorption spectroscopy (XAS), which can detect more information in amorphous catalysts. Generally, the elemental oxidized state, coordination number, and chemical environment can be analyzed by the peak shape, position, intensity, and other characteristics of the XANES spectrum. For instance, the valence states of Mn in the original and oxygen-vacant samples were revealed by XANES, and they range between 3+ and 4+[103]. Compared with XANES, extended X-ray absorption fine structure (EXAFES) can provide more detailed information on interatomic distances and coordination numbers. The structural information on amorphous catalysts can be obtained by analyzing the background noise and vibrational characteristics in the EXAFES spectrum. For example, Pan et al. used XANES and EXAFES to explore the atomic coordination information in amorphous H-CrOx/C-500 [Figure 9B and C][104]. The abundant coordination defects and oxygen vacancies in H-CrOx/C-550 can be revealed by the Cr-O and Cr-Cr coordination bonds [Figure 9C], the fitting results and wavelet transforms (WT) of Cr K-edge EXAFS [Figure 9D]. Significantly, the intensity and oscillation amplitude decrease in XANES and EXAFS is associated with highly disordered/amorphous structures. The presence of functional groups and short-range ordered structures of amorphous catalysts can be identified by Fourier transform infrared spectroscopy (FTIR) and Raman spectroscopy, respectively. For instance, the peaks at 860 and 685 cm-1 correspond to Fe(NiMo)-O [Figure 9E], while the peaks at 457, 529, and 664 cm-1 are associated with the vibration of FeNi-O in Fe-Ni oxide [Figure 9F][105], demonstrating the formation of amorphous Fe-Mo-O/NF. The specific surface area of amorphous catalysts can be measured by the N2 adsorption-desorption curve. As displayed in Figure 9G, the specific surface areas of Cu nanoclusters/FeN4 composites deposited on N-doped graphene (Cu/Fe-NG) and NG catalysts are 303.7 and 542.6 m2 g-1[106], respectively. Extensive research has been done to experimentally characterize amorphous catalysts, but their detailed crystal structures are still lacking, resulting in the relationship between structure and performance remains unclear. Thus, developing advanced experimental characterizations to detect more information from amorphous catalysts is urgent. X-ray photoelectron spectroscopy (XPS) can characterize the chemical composition, surface chemical state and electronic structure of amorphous catalysts; monitor the surface changes during the reaction process and other aspects, which contributes to an in-depth understanding of amorphous catalysts in terms of their structure, surface reaction properties and catalytic performance. Electron energy loss spectroscopy (EELS) imaging, as a high-resolution, high-sensitivity, and non-destructive catalyst characterization technique, plays an important role in the study of the chemical composition, microstructure, electronic energy level distribution, and surface reactive sites of amorphous catalysts, which contributes to an in-depth understanding of amorphous catalysts. Based on the Cu valence state mapping of EELS spectral imaging, Chen et al. obtained that metallic Cu can be oxidized by SC CO2 into amorphous CuxO, forming a structure with a core of Cu and an amorphous shell of CuxO[107].
Theoretical characterizations
Typically, the complicated properties of amorphous catalysts offer difficulty in capturing full information through experiments. The insufficient experimental characterizations provide a research platform for the development of theoretical characterizing amorphous catalysts. Machine learning (ML) can acquire the most representative feature from numerous data by automatically extracting and selecting features, thus improving its identifiability[108,109]. Combining numerous experimental data and simulation calculations, a model can be built to predict the structure of amorphous catalysts while designing and optimizing the structure by an optimization algorithm. For instance, the formation of amorphous CuxO was analyzed via ML [Figure 10] and can be roughly divided into three steps: surface oxidation, O penetration, and O departing[107].
Figure 10. (A-F) The simulated mechanism for the amorphization. (Reproduced with permission[107]. Copyright 2023, Nature).
DFT plays an important role in characterizing amorphous catalysts, and the main roles are summarized as follows. (1) The optimization of the potential energy surface can predict amorphous catalyst structures, such as atomic positions, crystal configuration, local environment, etc.[110]; (2) The movement of atoms and molecules in amorphous catalysts can be stimulated by molecular dynamics (MD) and other methods[111], thus revealing the dynamic process of catalytic reactions; (3) The catalytic activity of amorphous catalysts can be predicted by calculating the surface energy, adsorption properties, and energy-band structures; and (4) The electronic principles of amorphous catalysts in catalytic reactions can be revealed by calculating their electronic structural properties, such as electron density, energy band structure, density of states, etc. For example, He et al. modeled the formation mechanism of 2D amorphous PtSex by using ab initio MD (AIMD) simulations based on DFT[112]. The PtSe2 crystal structure with a PtSex surface was obtained by sequentially removing Se atoms from the PtSe2 monolayer and then calculating the selenium-depleted PtSex monolayers to create an amorphous structure via AIMD. Moreover, the combination of ML and DFT can explore the complex structure-activity relationship in binary or ternary multi-metal (hydro)oxide amorphous catalysts[113].
MODIFICATION STRATEGIES OF AMORPHOUS CATALYSTS
Most amorphous catalysts feature the merits of numerous low-coordinating atoms, more randomly oriented unsaturated bonds, and flexible local structures, thus delivering excellent electrocatalytic activity. However, the large-scale applications of amorphous catalysts remain challenging. Therefore, numerous strategies have been developed to modify their compositions, defects, and surface properties, which are crucial for electrocatalytic reactions. The regulation strategies of amorphous catalysts can be summarized as structural design, composition tuning, surface modification, interfacial engineering, and electrochemical modification.
Structural design
An effective way to tune electrocatalytic properties is to develop innovative structural designs. The designed distinct structures can manipulate the inherent features of catalysts, such as electronic structures and mass transfer. At the same time, amorphous metal oxides with oxygen vacancies offer unique advantages for electrocatalysis. They provide high surface area, enhanced mass transport properties and tunable electronic structure, which facilitates efficient electrocatalytic processes[114]. For instance, single Ga atoms were distributed in the oxygen vacancies of lotus-root-like a-TiO2 nanofibers (Ga-SA/a-TiO2) [Figure 11A-C] to improve their selectivity for NRR[98]. The inset SAED indicates the amorphous nature of a-TiO2 and
Figure 11. (A) SEM and (B) TEM images of a-TiO2. (C) TEM image of Ga SA/a-TiO2 nanofibers. (D) EPR spectra of Ga SA/a-TiO2 nanofibers. (E) NH3 yields and FEs of Ga SA/a-TiO2 nanofibers at different potentials for 2 h (Reproduced with permission[98]. Copyright 2022, American Chemical Society). (F) TEM images and SAED pattern of NiFe-LDH@Fe-MOF/IF-2. (G) HRTEM image of NiFe-LDH@Fe-MOF/IF-2. (H) LSV curves of various samples. (I) Corresponding Tafel plots. (Reproduced with permission[115]. Copyright 2021, Elsevier).
Tuning the self-supported electrode structures can improve their catalytic properties. This can be mainly attributed to (1) Self-supporting system electrocatalytic materials are directly grown or anchored on the conductive substrate through a certain process without the need for adhesives, thus avoiding its inhibitory effect on the conductive ability of the material; (2) Most self-supporting substrates themselves have good electrical conductivity, which can effectively improve the electron transmission of electrocatalytic materials with poor conductivity and promote the effective transmission of carriers during the catalytic process; (3) A strong interaction can be formed between the active material and the substrate. The strong contact interface between the electrocatalytic material and the self-supporting substrate can efficiently transport electrons and reduce the reaction barrier; and (4) Due to the strong contact between the electrocatalytic material and the self-supporting substrate, it avoids the shortcoming of easily falling off during the catalytic process using binders, so it shows good catalytic stability. For example, a self-supported amorphous CoMoO4 nanowire array on Ti mesh (CoMoO4 NWA/Ti) was produced by a simple two-step hydrothermal approach[116], which exhibits excellent HER activity and stability in 1 M KOH solution. Moreover, amorphous
Figure 12. (A) SEM and (B) HRTEM images of SA-MoO2/Ni3(PO4)2/NF. (C) ECSA estimated by double-layer capacitance measurements. (D) Nyquist plots. DFT calculations: (E) Free energy of hydrogen adsorption. (F) Model of MoO2 (-111) and (G) model of SA-MoO2 (-111). (H) Energy barrier of water dissociation. (Reproduced with permission[99]. Copyright 2023, Wiley-VCH).
Composition tuning
The composition regulation can optimize the electronic structure and surface chemistry of the amorphous catalysts, generating active sites that facilitate electron transfer and improve conductivity, thus improving their catalytic activity and stability. The composition modification strategies include alloying, doping, and compounding. For instance, introducing Co2+ to amorphous MoSX creates a Mo-S-Co structure[117], which increases active sites from unsaturated Mo atoms and S atoms, making it easier for electrons to migrate between the surface of the catalyst and the active sites, increasing the rate of electron transfer, and then improves its intrinsic electrocatalytic activity. Moreover, a novel amorphous P-doped MoS2 nanocomposite was prepared by a facile hydrothermal method[118]. The obtained amorphous P-doped MoS2 catalyst delivers better catalytic activity and stability than that of the crystalline MoS2 in acidic electrolytes. The Fe element was doped in amorphous Ni-P-B-O (NPBO) nanocages to prepare Fe-NPBO (FNPBO) catalysts and their amorphous feature was revealed via XRD patterns [Figure 13A][119]. The FNPBO, as an OER catalyst, exhibits a smaller overpotential and a smaller Tafel slope than that of NPBO [Figure 13B]. Amorphous NiWO4 and Fe-doped NiWO4 powders were prepared by the solution without or with Fe(NO3)3·6H2O, respectively [Figure 13C][120]. They show disordered structures [Figure 13D-G], and the electronic structure of NiWO4 was tuned by Fe3+ doping [Figure 13H], improving electron transfer efficiency and further enhancing catalytic performance. The OER overpotential of the synthesized Fe-doped amorphous NiWO4 exhibits a 90 mV drop than that of amorphous NiWO4 at 10 mA cm-2 in 1.0 M KOH solution [Figure 13I]. The specific surface area of amorphous N, P, and F triple-doped CoFe2O4 was enlarged by attaching ultrafine MoS2 nanoclusters on its surface. Since oxygen vacancies can affect the adsorption energy of intermediates, they change the reaction pathways, lower the reaction energy barriers, and improve the catalytic activity and selectivity of electrocatalysts. To further improve their activity, oxygen vacancies were created by using ionic liquids as dopants; thereby, the oxygen-vacancy-rich MoS2/NPF-CoFe2O4 (MNC) catalysts were prepared[121]. As shown in Figure 13J, the absence of diffraction peaks in the XRD pattern signifies the amorphous feature of MNC. The numerous oxygen vacancies, amorphous structure, large surface area (148.9 m2 g-1), and active sites (such as N-Fe, N-Co, etc.) in MNC contribute to its superior OER performances, showing an overpotential of 250 mV and a Tafel slope of 41 mV dec-1 at 10 mA cm-2
Figure 13. (A) XRD patterns of the FNPBO products with different ratios of Fe to Ni. (B) LSV curves of different samples. (Reproduced with permission[119]. Copyright 2019, American Chemical Society). (C) The synthesis of systematic amorphous NiWO4 and Fe-doped amorphous NiWO4. (D) HRTEM and (E) SAED of NiWO4. (F) HRTEM and (G) SAED of Fe-doped NiWO4. (H) Possible crystal field study of NiWO4 with or without Fe3+ doping. (I) Backward LSV curve of NiWO4, Fe-doped NiWO4, annealed NiWO4, and annealed Fe-doped NiWO4 at a scan rate of 5 mV/s in 1 M KOH. (Reproduced with permission[120]. Copyright 2023, American Chemical Society). (J) XRD patterns of the prepared catalysts. (K) N2 adsorption-desorption isotherm and pore-size distribution (inset) of the MNC. (L) OER polarization curves without iR-compensation. (M) Tafel slopes. (N) The elementary steps for the OER process catalyzed by various catalysts. (Reproduced with permission[121]. Copyright 2018, WILEY-VCH).
Per the above analysis, composition tuning can significantly improve the catalytic properties of catalysts. On the one hand, the adjustment of the catalyst composition can expand the reaction types, thus realizing various reactions via catalyst design and preparation. Catalysts with varied compositions have distinct chemical characteristics, surface structures, and crystal structures that allow them to be applied to a range of reactants and reaction conditions while enhancing catalytic activity. On the other hand, tuning the ratio and distribution of catalyst components can regulate crystal structure, coordination environment, electronic structure, and other surface properties, thus exposing more active sites while optimizing their adsorption and reaction properties, which, in turn, reduces charge transport paths and losses, accelerates charge transfer efficiency, and improves the conductivity of the material itself. Finally, the tuned composition can limit phase transitions and lattice flaws occurring in catalysts and then reduce catalyst deactivation during the reaction process and exhibit excellent catalytic stability.
Surface modification
The factors of the electronic structure and surface acid-base properties that affect the catalytic reactions of amorphous catalysts can be controlled by surface modification. For example, the microstructure of amorphous FNPBO can be adjusted by various Fe/Ni ratios [Figure 14A-F][119]. The microstructure changes from nanocages to NSs as the Fe/Ni ratio in FNPBO increases, thus altering its OER performance. The optimized FNPBO (Fe6.4Ni16.1P12.9B4.3O60.2) delivers excellent OER activity of 236 mV overpotential and
Figure 14. TEM of FNPBO with different Fe/Ni ratios: (A) Fe0.3Ni28.6P6.8B8.7O55.6; (B) Fe4.0Ni20.9P11.6P4.5O58.9; (C) Fe6.4Ni16.1P12.9B4.3O60.2 (the insert is SAED pattern); (D) Fe10.2Ni12.0P12.3B4.9O60.6; (E) Fe10.0Ni5.5P15.5B5.8O63.1; and (F) Fe16.8Ni6.1P9.3B7.7O60.1. (G) Tafel slope-potential plots of the Ni33.3B14.7O52.0, Ni33.7P3.2B10.2O52.9, FNPBO catalysts, and commercial RuO2. (H) Durability test of Fe6.4Ni16.1P12.9B4.3O60.2 nanocages at 236 mV. (Reproduced with permission[119]. (Copyright 2019, American Chemical Society).
Interfacial engineering
The optimization of the interaction between amorphous catalysts and reactants, the improvement of the catalyst surface activity, and the regulation of surface active centers and adsorption sites can be realized by interfacial engineering, thus selectively modulating various reactions. Meanwhile, the microcrystalline regions present in the amorphous catalysts can influence the electrocatalytic performance of the materials, which is due to the fact that the coexistence of the crystalline and amorphous phases can provide a richer surface structure, increase the density of active sites, and improve the reactivity of the catalysts; modulate the surface energy of the catalysts, which makes the reactants easier to be adsorbed and transformed; improve the charge transport performance of the catalysts, reduce the resistance, and increase the electron transport rate, thus accelerating the reaction rate; and increase the stability and resistance to loss of activity, which prolongs the service life of the catalysts. For instance, a new amorphous/crystalline CrOx-Ni3N with heterostructure was grown on NF via the combination of hydrothermal and nitridation[123]. The DFT calculations indicate that the electronic structure modulation at the CrOx-Ni3N interface is conducive to optimizing H2O dissociation (∆GH-OH) and H adsorption (∆GH), thus, in turn, contributing to excellent and stable HER properties [Figure 15A]. Meanwhile, CrOx-Ni3N delivers a higher electron density near the Fermi energy level than that of Ni3N, suggesting its improved conductivity [Figure 15B]. The CrOx-Ni3N heterostructure shows nanoparticles decorated NS structure [Figure 15C] and exhibits mixed phases; the nanoparticles are crystalline Ni3N, while the NSs are amorphous CrOx [Figure 15D]. The abundant oxygen vacancies and amorphous/crystalline interfaces endow CrOx-Ni3N catalysts with more active site exposure and increased intrinsic activity, resulting in excellent HER performances. Moreover, the
Figure 15. (A) The calculated Gibbs free energy of OH-H and H adsorption for the Ni3N, CrOx, and CrOx-Ni3N. (B) The total PDOSs of the Ni3N and CrOx-Ni3N. (C and D) TEM characterizations of CrOx-Ni3N. (Reproduced with permission[123]. Copyright 2022, Wiley-VCH). (E) Schematic illustration of the overall water splitting process. Amorphous CFOH/COH catalyst exhibits much better performances than other as-synthesized A-F or A-C catalysts. (Reproduced with permission[124]. Copyright 2020, Elsevier). (F and G)TEM and HRTEM of NFSe@NFOH/NF. (I and J) Corresponding FFT patterns of the selected regions marked by purple and pink squares, respectively. (H) Lattice spacing corresponds to the selected white region. (Reproduced with permission[125]. Copyright 2023, Elsevier).
In short, catalyst interfacial engineering can create heterogeneous structures forming microcrystalline regions and significantly increase catalytic activity. The first reason is that the formed heterogeneous structure exposes more active sites and expands the reaction boundary area, thus enhancing the activity at the electrolyte-electrode interfaces. Another reason is that heterostructures can modulate catalyst electronic structures and then improve catalytic activity. The heterostructure interfaces of different materials can provide various lattice structures, change local potentials, modulate electronic states, alter energy levels, and tune catalyst surface active sites, thereby enhancing the charge transfer rate and improving the material conductivity. Finally, the formed amorphous interfaces reduce local stress and dislocation density in crystals, decrease the formation and diffusion of lattice defects, and prevent surface oxidation and corrosion, thus improving the adaptability and long-term stability of catalysts.
Electrochemical modification
The potentials or currents are conducted to electrochemical modulate amorphous catalysts, thus activating the catalyst surface or modulating the electrocatalytic process. The introduction of activatable functional groups, such as hydroxyl and carboxyl groups, on the surface of amorphous catalysts by electrochemical methods can increase the active sites of the catalysts and improve the reactivity; optimize the paths and rates of electron transport and improve the conductivity. For example, the amorphous high-entropy CoNiFeMn phosphorus oxide (CNFMPO) [Figure 16A] was obtained by a simple low-potential electrodeposition method[126]. The HER-activated CNFMPO undergoes surface reconstruction; during the activation process, the NS layer gradually shrinks and thickens for surface dissolution and redeposition of metal salts. Similarly, OER-activated CNFMPO undergoes surface reconstruction; the surface elements, phosphite, and phosphate at high valence experience dissolution and redeposition, resulting in the NSs gradually transforming into fine nanoparticles and reconstructing into oxides or (oxy)hydroxides. Compared with the reference samples, these structural changes endow the activated CNFMPO catalyst electrode with a faster electron transfer rate, an increased electrochemically active surface area, and more active sites, delivering excellent HER and OER properties in 1 M KOH solution [Figure 16B and C]. Moreover, the amorphous small Sn(HPO4)2 (SnP) nanoparticles were prepared by Cheng et al. from a hydrothermal method[127]. Placing SnP in a
Figure 16. (A) TEM images of CNFMPO (inset is SAED). (B) EIS Nyquist plots of HER-activated electrodes. (C) Plots of capacitive current as a function of scan rates for OER-activated electrodes. (Reproduced with permission[126]. Copyright 2024, Elsevier). (D) A proposed in situ derivatization process. (E) HRTEM images of SnP after CO2RR. (F-I) Adsorption configurations of CO2 on the surfaces of SnO (F), SnO-PO4 (G), and P-O-Sn (H). (I) Free energy diagram of CO2RR to HCOOH. (Reproduced with permission[127]. Copyright 2023, Elsevier).
From the above examples, the main reasons for how electrochemical modification improves electrocatalyst catalytic activity can be summarized as follows. (1) Regulating electronic states: The applied voltage can tune the surface-distributed electrons of electrocatalysts, the electron density of active sites, and the adsorption characteristics of reactants. Generally, applying a positive potential can expose more surface active sites and improve the adsorption/activation efficiency of reactants, thus enhancing the catalytic activity; (2) Improvement of charge transport: Modulation of the electron transport properties of amorphous catalysts to reduce resistance, increase electron transport rate, and enhance conductivity; (3) Promoting reaction kinetics: The potential of reactants can be adjusted by applying an external potential, and then changing the activation energy and reaction rate constant. Commonly, the applied proper potential facilitates high-energy intermediates or atomically active states to form on the catalyst surfaces, thus lowering the activation energy of reactants and increasing the reaction rate; and (4) Modulating surface adsorption energy: The exerted applied potential can change the catalyst surface distributed charge and regulate the surface reactant adsorption strength. The increased adsorption sites and moderate adsorption strength can optimize the reactant adsorption and activation states on catalyst surfaces, finally improving catalytic activity.
APPLICATIONS OF AMORPHOUS CATALYSTS IN ELECTROCATALYSIS
The large specific surface area, high catalytic activity, good electron conductivity, and structural durability enable amorphous materials to be widely applied in electrocatalysis. As illustrated in Figure 17, the main research progress of amorphous materials in electrocatalysis is briefly summarized, and their applications in HER, OER, ORR, Co2RR, and NRR are mainly introduced as follows[128-139].
HER
As a crucial half-reaction in electrocatalytic water splitting, HER plays a pivotal role in sustainable energy conversion and storage fields[140-142]. This reaction involves a two-electron transfer process, and its pathways can be classified as the Volmer-Heyrovsky process (always used in electrochemical desorption mechanism) and the Volmer-Tafel process (commonly applied in recombinant desorption mechanism). The formation of hydrogen intermediate (H*) is expressed in the following Equations (3-8), which are substantially influenced by the pH of the electrolyte[141].
In acidic media:
In alkaline media:
The HER mechanism typically contains the steps of electron transfer, atomic rearrangement, intermediate species formation and dissociation, etc. In detail, the hydrogen ions on the cathode surface receive electrons and form hydrogen atoms (H*), and then, two H* integrate to form H2 molecules[143]. The occurrence of HER needs to introduce catalysts that can reduce activation energy and accelerate reaction kinetics. In the currently studied electrocatalysts, amorphous catalysts feature the advantages of high activity, uniform loading, low catalyst deactivation rate, and tunability, receiving wide applications in HER.
For example, amorphous/crystalline Rh(OH)3/CoP heterostructure was constructed to catalyze HER in the entire pH range [Figure 18A][144]. In 1.0 M KOH solution, Rh(OH)3/CoP delivers a lower overpotential of
For example, amorphous/crystalline Rh(OH)3/CoP heterostructure was constructed to catalyze HER in the entire pH range [Figure 18A][144]. In 1.0 M KOH solution, Rh(OH)3/CoP delivers a lower overpotential of
Figure 18. (A) Synthesis illustration of the Rh(OH)3/CoP. Electrocatalytic HER performance in 1 M KOH solution. (B) HER polarization curves, (C) Tafel plots. (Reproduced with permission[144]. Copyright 2023, Wiley-VCH). (D) H-metal distance contribution analysis as the function of ΔGH. (Reproduced with permission[147]. Copyright 2023, Elsevier). (E) LSV polarization curves for the HER of different catalysts. (Reproduced with permission[148]. Copyright 2021, Elsevier). (F) The electrocatalytic HER properties of the recent amorphous catalysts.
OER
For water splitting, another key half-reaction is OER. However, the four-electron transfer involved in OER leads to a great challenge in improving overall water-splitting kinetics. The commonly accepted OER mechanism at acidic (Equations 9-12) or alkaline (Equations 13-16) conditions includes the following four steps, respectively[157]. The OER operates in acidic or alkaline solutions, both involving the formation of OH*, O*, and OOH* intermediates. The specific reaction pathways and kinetics of OER highly depend on the binding strength of M-OH*, M-O*, or M-OOH*[158].
In acidic media:
In alkaline media:
Effective electrocatalysts play a crucial role in driving water splitting. As previously reported electrocatalysts, amorphous catalysts show unparalleled advantages in electrocatalyzing OER and are receiving increasing attention. For instance, hollow multichannel amorphous NiFe nanoreactors (NiFe-ANR) were produced as OER electrocatalysts[159], and their performances overmatch those of crystalline nanoreactor and amorphous non-nanoreactor catalysts. The amorphous structure enables NiFe-ANR to oxidize and form high-valent reactive species; meanwhile, numerous microcrystalline interfaces provide more active sites. Furthermore, the nanoreactor structure causes a localized high hydroxide concentration in NiFe-ANR and effectively promotes OER. As a result, the overpotential of NiFe-ANR (228 mV) is 118 mV lower than NiFe-CNR and 75 mV lower than NiFe-ANR at 10 mA cm-2. A pervasive top-down strategy was developed by Liu et al. to prepare amorphous oxide materials by low-temperature treating chalcogenide hydroxides [Figure 19A], which is capable of amorphizing a variety of unitary, binary, and ternary chalcogenide hydroxides[160]. The current density of the obtained ternary FeCoSn(OH)6-300 amorphous oxide reaches 178 mA cm-2 at 1.6 VRHE and stably operates for 200 h at 100 mA cm-2 [Figure 19B and C], which surpasses many previously reported OER electrocatalysts. DFT calculations reveal that amorphization strongly promotes the p-d hybridization between Co and Fe sites, significantly lowering the potential barrier of the rate-determining step that converses OH* to O* in OER [Figure 19D and E]. The structural evolution of FeCo-POx from crystalline to amorphous can be regulated by the precursor anion etching time [Figure 19F][161]. The gained amorphous FeCo-POx exhibits a low overpotential of 270 mV at 10 mA cm-2 and almost no degradation operating for
Figure 19. (A) Schematic illustration for the structural evolution of CoSn(OH)6 during amorphization. (B) The current densities of MCoSn(OH)6 and MCoSn(OH)6-300 in O2-saturated 1.0 M KOH solution. (C) Continuous OER chronopotentiometry test for FeCoSn(OH)6-300 at 100 mA cm-2. (D) The PDOS of FeCoSn(OH)6-300. (E) The energetic trend of OER at U = 0 V. (Reproduced with permission[160]. Copyright 2022, Nature). (F) XRD patterns of Fe-POx 0.5~1.5 h and A-Fe-POx. (G) Chronopotentiometric curves of the long-term stability of electrodes at 10 mA cm-2 without iR compensation (Inset: polarization curves initial and after 2,400 CV). (Reproduced with permission[161]. Copyright 2023, Elsevier). (H) The recent reports of amorphous electrocatalyst properties in OER.
ORR
The inverse reaction of OER is ORR, which is extremely important in the applications of fuel cells, metal-air batteries, sensors, and other electrochemical devices. The conversion efficiency from chemical to electrical in energy conversion devices strongly depends on ORR. Typically, there are two kinds of reaction pathways of ORR. One is reduced O2 to H2O or OH- via a four-electron transfer pathway in acidic and alkaline media, respectively[170]. Another pathway is reduced O2 to H2O2 (acidic solution) or HO2- (alkaline solution) via a two-electron transfer. Taking a four-electron transfer as an example, ORR can be expressed by the following half-reaction (Equations 17 and 18)[171].
In acidic media:
In alkaline media:
The common ORR catalysts, such as Pt-group metals and transition metal-based materials, need further optimization to realize practical applications. Amorphous ORR electrocatalysts come into being on this basis and attract marvelous concern. We summarized the recent amorphous electrocatalysts in ORR, as shown in Figure 20A[172-181]. Oxygen vacancies in amorphous catalysts play an important role in the ORR: they can provide reactive sites and act as electron transport media, participating in the electron transfer and energy level regulation on the catalyst surface, which affects the reaction rate and selectivity; at the same time, the active oxygen vacancies can react with oxygen or oxides to form intermediates of redox reactions and promote the reaction. For example, amorphous mullite SmMn2O5-δ nanoparticles with controllable oxygen vacancy concentration were prepared to catalyze ORR[103]. Moderate oxygen defects endow
Figure 20. (A) The recent amorphous electrocatalysts in ORR. (B) Schematic of synthesis for Fe subnanocluster/3D-C. (C) Mass activity for Pt/C, Fe NP/3D-C, and Fe sub-nano-cluster/3D-C. (D) methanol tolerance tests for Fe sub-nano-cluster/3D-C. (Reproduced with permission[173]. Copyright 2019, American Chemical Society). (E) LSV curves of as-prepared catalysts and Pt/C. (F) Computational models, O2 adsorption configurations, and corresponding adsorption energies for FeN4 and CuNx-FeN4 (X = 2,3,4). (Reproduced with permission[106]. Copyright 2020, American Chemical Society).
Amorphous Cu/Fe-NG was formed via vacuum annealing and exhibited excellent ORR activity
CO2RR
CO2RR is a transformational process designed to use renewable energy to convert greenhouse gas CO2 into valuable carbon-based products. This reaction provides a promising way to combat climate change, develop sustainable energy, and achieve carbon-neutral energy systems. However, CO2RR involves a variety of products, such as C1 products (CO, HCOOH, CH4, etc.), C2 products (C2H4, C2H6, C2H3OH, C2H2OH, etc.), and C3+ products (C3H6, C3H8O, etc.). The reaction formulas and related theory potentials for the listed
The multiple products endow CO2RR with complex mechanisms, which can be ascribed to the formation of various reaction intermediates (*CO, *CCH, etc.) and different reaction pathways. Therefore, it is urgent to develop high-activity and high-selectivity electrocatalysts for CO2RR. Amorphous CO2RR electrocatalysts feature the merits of high activity, good selectivity, and stability, thus drawing increasing concern. We concluded the recent CO2RR properties of amorphous electrocatalysts in Table 2.
CO2RR properties of amorphous electrocatalysts in the recent literature
Materials | Main products | Electrolytes | FE (%) | Potentials (vs. RHE) | Current density (mA/cm2) | Ref. |
a-MnOx-H | CO | 0.1 M KHCO3 | 94.8 | -0.62 V | 10.4 | [184] |
CuBi-100 | Formate | 0.5 M KHCO3 | 94.7 | -1.0 V | 12.8 | [185] |
a-Cu | HCOOH | 0.1 M KHCO3 | 37 | -1.4 V vs. Ag/AgCl | - | [186] |
C2H6O | 22 | |||||
H-InOx NRs | HCOOH | 0.5 M NaHCO3 | 90.2 | -0.8 V | 7.8 | [187] |
P-Cd|S | CO | 0.25 M K2SO4 | 88 | -0.8 V | 89.8 | [188] |
AgBi-500 | HCOOH | 0.1 M KHCO3 | 94.3 | -0.7 V | 12.52 | [189] |
For instance, amorphous copper nanoparticles exhibit excellent liquid product selectivity when used as
Figure 21. (A) The FE of the liquid products of a-Cu and c-Cu at each given potential for 2 h. (Reproduced with permission[186]. Copyright 2018, WILEY-VCH). (B) The distribution of C2+ products over R-8-Cu-12. (C) The plot of C2+ oxygenates partial current density vs. maximum C2+ oxygenates FE for various catalysts. (Reproduced with permission[107]. Copyright 2023, Nature). (D) HRTEM (the inset is FFT) image of CuBi-100. (E) FE of formate for CuBi-100 and CuBi-100a. (F) Partial current density of formate for two samples in all potentials range. (Reproduced with permission[185]. Copyright 2021, Elsevier).
NRR
NRR refers to the chemical process of converting N2 into organic nitrogen compounds. The main product of NH3 serves as a vital component in agriculture and industry. However, the thermodynamic stability of the N≡N bond and the competitive reaction of HER limit the NH3 yield and FE. The development of high-active NRR electrocatalysts is urgent. In the developed electrocatalysts for NRR, abundant surface active sites, high surface area, porous structure, and good electron conductive endow amorphous catalysts with excellent NRR performances. We concluded the recent amorphous electrocatalysts for NRR in Table 3.
NRR properties of amorphous electrocatalysts in the recent literature
Electrocatalyst | Electrolyte | NH3 yield | Potential (vs. RHE) | FE (%) | Ref. |
PdCoCu NSs | 0.1 M KOH | 60.68 µg h-1mgcat-1 | -0.05 V | 42.93 | [190] |
a-NiSb2O6-x nanofiber | 0.1 M Na2SO4 | 6.8 μg h-1 mg-1 | -0.3 V | 23.3 (-0.25 V vs. RHE) | [191] |
H-CrOx/C-550 | 0.1 M Na2SO4 | 19.10 μg h-1 mgcat-1 | -0.7 V | 1.4 | [104] |
Ni0.50Fe0.50B | 0.1 M KOH | 3.24 μg h-1 cm-2 | -0.3 V | 3.19 | [192] |
MoS3 | 0.5 M LiClO4 | 51.7 μg h-1 mg-1 | -0.3 V | 12.8 | [193] |
CoMoO4 | 0.1 M PBS | 30.2 μg h-1 mgcat-1 | -0.5 V | 3.8 | [194] |
BiNi | 0.1M Na2SO4 | 17.5 μg h-1 mgcat-1 | -0.6 V | 13.8 | [195] |
For example, amorphous NiSb2O6-x nanofibers (a-NiSb2O6-x) with Janus structure were proposed via the different but complementary electronic structures between d- and p- block elements [Figure 22A][191]. A high NH3 FE of 23.3% at -0.25 VRHE was obtained from a-NiSb2O6-x [Figure 22B]. Meanwhile, the yield of NH3 at -0.3 VRHE (6.8 μg h-1 mg-1) is much larger than that of NiO nanofibers (2.4 μg h-1mg-1,6.0%), Sb2O4 nanofibers (1.5 μg h-1 mg-1, 8.7%), and their crystal materials. Furthermore, a-NiSb2O6-x nanofibers can stably operate for 12 h [Figure 22C]. Compared to the crystalline counterpart, a-NiSb2O6-x exhibits a lower binding energy (-0.27 eV) and a strong adsorption capacity for N2 by the synergy between Ni and Sb atoms [Figure 22D], thus resulting in high activity for NRR. Amorphous PdCoM (M = Cu, Ag, Fe, Mo) with 2D porous NS architectures [Figure 22E] were prepared to reduce N2[190]. The amorphous PdCoCu delivers excellent NRR electrocatalytic activity with an NH3 yield of 60.68 µg h-1 mgcat-1 and an FE of 42.93% at
Figure 22. (A) Schematic diagram showing the process for the formation of a-NiSb2O6-x nanofiber. (B) NH3 yield and FE of a-NiSb2O6-x nanofiber at each potential. (C) Chronoamperometry curve of a-NiSb2O6-x nanofiber at 0.3 VRHE for 12 h. (D) Binding energies of different adsorption configurations of N2 on a-NiSb2O6-x, pc-NiSb2O6-x, and c-NiSb2O6-x, respectively. (Reproduced with permission[191]. Copyright 2022, Elsevier). (E) SEM image of PdCoCu NSs. (F) Histograms of average NH3 yields and corresponding FE of PdCoCu NSs at different potentials. (G) Proposed reaction mechanism for the reduction of N2 to NH3. (Reproduced with permission[190]. Copyright 2023, Wiley-VCH).
SUMMARY AND PROSPECTS
This review summarized the recent advancements in classification, preparation, modification strategies, characterizations, and electrochemical applications of amorphous catalysts. Amorphous structures feature the merits of rich chemical compositions, high specific surface area, size effect, and structural flexibility, becoming a kind of potentially efficient, stable, and controllable electrocatalysts. However, the large-scale applications of amorphous catalysts face several challenges, resulting in their further research should focus on the following aspects.
(1) Amorphous high entropy materials: Amorphous high-entropy catalysts are composed of multiple elements that form a highly disordered amorphous structure, which gives them abundant surface defects and active sites, thus enhancing their catalytic activity and selectivity. Their complex structures should be characterized by advanced characterization techniques, such as in-situ XPS and in-situ TEM, to better understand the formation and evolution of active sites in HEAs, which can lead to the design of more efficient electrocatalysts. Utilizing advantage of the adjustable composition of amorphous high-entropy catalysts and their excellent corrosion resistance and thermal stability, the catalytic performance can be precisely regulated by adjusting the composition and preparation process, which can satisfy the needs of different catalytic reactions and be applied to complex industrial catalytic environments. By taking advantage of the adjustable composition of amorphous high-entropy catalysts and their excellent corrosion resistance and thermal stability, the catalytic performance can be precisely regulated through the modulation of composition and preparation process, which can satisfy the needs of different catalytic reactions and make it applicable to complex industrial catalytic environments.
(2) Synthesis method: For the preparation methods of amorphous materials, such as rapid solidification and sputtering, which always need high temperature, high pressure, and complex processes, leading to poor controllability and high cost. Thus, developing simple and efficient methods to prepare amorphous catalysts is urgent.
(3) Large-scale preparation of amorphous materials: The development of cost-effective and scalable synthesis methods for catalysts is crucial for their practical use in industrial applications. Therefore, researchers should aim to develop efficient and controllable preparation processes that can be applied on a large scale to promote the large-scale production of amorphous catalysts. In addition, integration with advanced manufacturing technologies, such as continuous flow synthesis, microfluidics, and additive manufacturing, should be considered to improve the efficiency of amorphous catalyst production.
(4) Size effect: The high specific surface area of amorphous materials generates a microscopic size effect that extremely influences catalytic properties. Deeply revealing and precisely regulating the size effect can endow amorphous catalysts with better catalytic performances.
(5) Characterizations: The disordered feature induces the characterizations of amorphous catalysts, with remaining challenges in surface structure and active site identification. Advanced techniques of theory and experiments need to be developed to reveal the relationship between amorphous structure and catalytic activity. Meanwhile, the dynamic evolution of amorphous structures in operating urgent needs to be clarified via in-situ and atomic-scale characterizations, such as in-situ FTIR, in-situ XAS, in-situ TEM, MD, etc.
(6) Catalytic activity: A decisive factor for an effective catalyst, but facing the challenges of unpredictable nature and variation in amorphous catalysts. To address these issues, ML and artificial intelligence need to be developed to analyze and model large-scale experimental data. The relationship between structure and catalytic performances in amorphous catalysts can be revealed, thus guiding the design of reliable and predictable strategies for tuning catalytic activity.
(7) Coexistence of amorphous/crystalline catalyst: The coexistence of amorphous and crystalline states of catalysts may provide unique performance advantages. Amorphous structures usually have higher surface area and active sites, while crystalline structures may have better stability and conductivity. Through careful design and combining the advantages of both, more efficient catalytic performance can be achieved. Additional integration of advanced preparation techniques and materials simulation tools is pivotal in understanding the structure-property relationships of amorphous and crystalline coexisting materials. This will lead to the precise design of materials with specific structures and functions for specific catalytic reactions.
(8) Operation stability: The short-range ordered amorphous structures undergo structural changes and transform into crystalline structures during the long-term catalytic reactions, reducing catalytic activity and stability. Addressing this challenge is urgent for designing highly efficient amorphous catalysts. The usually used methods are tuning alloy compositions and surface structures; however, these approaches face several inherent issues. Therefore, novel strategies are urgently needed to develop from experimental and theoretical results, enabling amorphous catalysts to operate long-term.
(9) Catalytic mechanism: Compared with crystalline catalysts, the absence of well-defined long-range ordered structures in amorphous materials induces more complex catalytic mechanisms. Advanced characterization techniques and theoretical methods need to be developed and to reveal the catalytic mechanism of amorphous catalysts, thus guiding their rational design and optimization.
In light of the above analysis, comprehensively understanding the basic principles of amorphous catalysis and exploring new synthesis strategies are key for developing highly active and stable amorphous catalysts. Importantly, sustainability concepts need to be considered in the design and preparation of amorphous catalysts, thus minimizing environmental impact and promoting sustainable energy transition. Meanwhile, strengthening the interdisciplinary cooperation of materials science, electrochemistry, and catalysis can drive the development and applications of amorphous catalysts. We hope this review can elucidate the huge potential and current research status of amorphous structures in electrocatalysis. Moreover, this review gives a platform to understand the role, performance, and challenges of amorphous catalysts in electrocatalysis. Finally, it provides a reference for the design of high-active and stable amorphous catalysts, along with insights into their practical applications.
DECLARATIONS
Authors’ contributions
Conceptualization, investigation, and writing-original draft: Yu Z, Sun Q
Writing-review & editing: Zhang M, Zhang L, Yang H, Chen Y, Guo J
Writing-review & editing, supervision, and funding acquisition: Zhang M, Zhang Z, Yu Z, Jiang Y
Availability of data and materials
Not applicable.
Financial support and sponsorship
This study was financially supported by the National Natural Science Foundation of China (No. 52102291, No. 52271011, and No. 51701142), Structure design and mass preparation of high stability and low-cost PEM hydroelectrolysis non-iridium catalyst (KC22453).
Conflicts of interest
All authors declared that there are no conflicts of interest.
Ethical approval and consent to participate
Not applicable.
Consent for publication
Not applicable.
Copyright
© The Author(s) 2024.
REFERENCES
1. Yang QR, Fan QN, Peng J, Chou SL, Liu HK, Wang J. Recent progress on alloy-based anode materials for potassium-ion batteries. Microstructures 2023;3:2023013.
2. Yu Z, Sun Q, Li H, et al. Tuning single-phase medium-entropy oxides derived from nanoporous NiCuCoMn alloy as a highly stable anode for Li-ion batteries. Rare Met 2023;42:2982-92.
3. Zhao SM, Wang BS, Zhu N, Huang Y, Wang F. Dual-band electrochromic materials for energy-saving smart windows. Carbon Neutralization 2023;2:4-27.
4. Zhang Z, Xie G, Chen Y, et al. Regulating the intrinsic electronic structure of carbon nanofibers with high-spin state Ni for sodium storage with high-power density. J Mater Sci Technol 2024;171:16-23.
5. Yan L, Zong L, Sun Q, et al. Phase separation-hydrogen etching-derived Cu-decorated Cu-Mn bimetallic oxides with oxygen vacancies boosting superior sodium-ion storage kinetics. J Energy Chem 2023;80:163-73.
6. Chen Y, Sun H, Guo J, et al. Research on carbon-based and metal-based negative electrode materials via DFT calculation for high potassium storage performance: a review. Energy Mater 2023;3:300044.
7. Abdelhafiz A, Wang B, Harutyunyan AR, Li J. Carbothermal shock synthesis of high entropy oxide catalysts: dynamic structural and chemical reconstruction boosting the catalytic activity and stability toward oxygen evolution reaction. Adv Energy Mater 2022;12:2200742.
8. Li L, Dai X, Lu M, et al. Electron-enriched single-Pd-sites on g-C3N4 nanosheets achieved by in-situ anchoring twinned Pd nanoparticles for efficient CO2 photoreduction. Advanced Powder Materials 2024;3:100170.
9. Khan I, Baig N, Bake A, et al. Robust electrocatalysts decorated three-dimensional laser-induced graphene for selective alkaline OER and HER. Carbon 2023;213:118292.
10. Li H, Du H, Luo H, Wang H, Zhu W, Zhou Y. Recent developments in metal nanocluster-based catalysts for improving photocatalytic CO2 reduction performance. Microstructures 2023;3:2023024.
11. Wang Y, Wang D, Li Y. Atom-level interfacial synergy of single-atom site catalysts for electrocatalysis. J Energy Chem 2022;65:103-15.
12. Li Y, Zhang J, Chen Q, Xia X, Chen M. Emerging of heterostructure materials in energy storage: a review. Adv Mater 2021;33:e2100855.
13. Yang Y, Li P, Zheng X, et al. Anion-exchange membrane water electrolyzers and fuel cells. Chem Soc Rev 2022;51:9620-93.
14. Li C, Zhang Z, Chen Y, et al. Architecting braided porous carbon fibers based on high-density catalytic crystal planes to achieve highly reversible sodium-ion storage. Adv Sci 2022;9:e2104780.
15. Li H, Chang SH, Zhang MM. Research progress on properties tuning and products of Cu-based catalyst in electrocatalytic CO2 reduction. Copper Eng 2023;6:38-50.
16. Zhang M, Wang J, Xue H, et al. Acceptor-doping accelerated charge separation in Cu2O photocathode for photoelectrochemical water splitting: theoretical and experimental studies. Angew Chem Int Ed 2020;59:18463-7.
17. Wang Y, Sun Y, Li H, et al. Controlled etching to immobilize highly dispersed Fe in MXene for electrochemical ammonia production. Carbon Neutralization 2022;1:117-25.
18. Kang S, Cheng J, Gao W, Cui L. Toward safer lithium metal batteries: a review. Energy Mater 2023;3:300043.
19. Liu H, Qin H, Kang J, et al. A freestanding nanoporous NiCoFeMoMn high-entropy alloy as an efficient electrocatalyst for rapid water splitting. Chem Eng J 2022;435:134898.
20. Wang Z, Berbille A, Feng Y, et al. Contact-electro-catalysis for the degradation of organic pollutants using pristine dielectric powders. Nat Commun 2022;13:130.
21. Li L, Wang P, Shao Q, Huang X. Recent progress in advanced electrocatalyst design for acidic oxygen evolution reaction. Adv Mater 2021;33:e2004243.
22. Wang Z, Richards D, Singh N. Recent discoveries in the reaction mechanism of heterogeneous electrocatalytic nitrate reduction. Catal Sci Technol 2021;11:705-25.
23. Ma W, He X, Wang W, Xie S, Zhang Q, Wang Y. Electrocatalytic reduction of CO2 and CO to multi-carbon compounds over Cu-based catalysts. Chem Soc Rev 2021;50:12897-914.
24. Chen M, Rao P, Miao Z, et al. Strong metal-support interaction of Pt-based electrocatalysts with transition metal oxides/nitrides/carbides for oxygen reduction reaction. Microstructures 2023;3:2023025.
25. Siu JC, Fu N, Lin S. Catalyzing electrosynthesis: a homogeneous electrocatalytic approach to reaction discovery. ACC Chem Res 2020;53:547-60.
26. Mchugh PJ, Stergiou AD, Symes MD. Decoupled electrochemical water splitting: from fundamentals to applications. Adv Energy Mater 2020;10:2002453.
27. Wang J, Cui W, Liu Q, Xing Z, Asiri AM, Sun X. Recent progress in cobalt-based heterogeneous catalysts for electrochemical water splitting. Adv Mater 2016;28:215-30.
28. Zhao C, Zhang X, Xu S, et al. Construction of amorphous CoFeOx(OH)y/MoS2/CP electrode for superior OER performance. Int J Hydrogen Energy 2022;47:28859-68.
29. Jin J, Ge J, Zhao X, Wang Y, Zhang F, Lei X. An amorphous NiCuFeP@Cu3P nanoarray for an efficient hydrogen evolution reaction. Inorg Chem Front 2022;9:1446-55.
30. Cheng D, Wang Z, Chen C, Zhou K. Crystalline/amorphous Co2P@FePO4 core/shell nanoheterostructures supported on porous carbon microspheres as efficient oxygen reduction electrocatalysts. Chem Mater 2019;31:8026-34.
31. Xu Y, Guo Y, Sheng Y, et al. Controlled boron incorporation tuned two-phase interfaces and Lewis acid sites in bismuth nanosheets for driving CO2 electroreduction to formate. J Mater Chem A 2023;11:18434-40.
32. Shi M, Bao D, Li S, Wulan B, Yan J, Jiang Q. Anchoring PdCu amorphous nanocluster on graphene for electrochemical reduction of N2 to NH3 under ambient conditions in aqueous solution. Adv Energy Mater 2018;8:1800124.
33. Wang X, Tian H, Yu X, Chen L, Cui X, Shi J. Advances and insights in amorphous electrocatalyst towards water splitting. Chin J Catal 2023;51:5-48.
34. Zhou Y, Fan HJ. Progress and challenge of amorphous catalysts for electrochemical water splitting. ACS Mater Lett 2021;3:136-47.
35. Zhai W, Sakthivel T, Chen F, Du C, Yu H, Dai Z. Amorphous materials for elementary-gas-involved electrocatalysis: an overview. Nanoscale 2021;13:19783-811.
36. Zhang C, Wang SY, Rong JF, Mi WL. Amorphous catalysts for electrochemical water splitting. China Pet Process Pe 2022;24:1-13. Available from: http://www.chinarefining.com/EN/Y2022/V24/I2/1 [Last accessed on 10 Apr 2024].
37. Wang H, Qi J, Yang N, et al. Dual-defects adjusted crystal-field splitting of LaCo1-xNixO3-δ hollow multishelled structures for efficient oxygen evolution. Angew Chem Int Ed 2020;59:19691-5.
38. Anantharaj S, Noda S. Amorphous catalysts and electrochemical water splitting: an untold story of harmony. Small 2020;16:e1905779.
39. Dan H, Chen L, Li Z, He X, Ding Y. Preparation of amorphous ZrO2 powders by hydrothermal-assisted sol-gel method. Inorg Chem Commun 2022;138:109272.
40. Liu H, Tariq NUH, Han R, et al. Development of hydrogen-free fully amorphous silicon oxycarbide coating by thermal organometallic chemical vapor deposition technique. J Non-Cryst Solids 2022;575:121204.
41. Wu Y, Zhang Z, Xu K, et al. A study on the formation conditions of amorphous nickel-phosphorus (Ni-P) alloy by laser-assisted electrodeposition. Appl Surf Sci 2021;535:147707.
42. Olowoyo JO, Kriek RJ. Recent progress on bimetallic-based spinels as electrocatalysts for the oxygen evolution reaction. Small 2022;18:e2203125.
43. Kumar A, Bhattacharyya S. Porous NiFe-oxide nanocubes as bifunctional electrocatalysts for efficient water-splitting. ACS Appl Mater Interfaces 2017;9:41906-15.
44. Nguyen TX, Liao Y, Lin C, Su Y, Ting J. Advanced high entropy perovskite oxide electrocatalyst for oxygen evolution reaction. Adv Funct Mater 2021;31:2101632.
45. Qiu H, Fang G, Gao J, et al. Noble metal-free nanoporous high-entropy alloys as highly efficient electrocatalysts for oxygen evolution reaction. ACS Mater Lett 2019;1:526-33.
46. Wang H, Liu R, Li Y, et al. Durable and efficient hollow porous oxide spinel microspheres for oxygen reduction. Joule 2018;2:337-48.
47. Fang L, Jiang Z, Xu H, et al. Crystal-plane engineering of NiCo2O4 electrocatalysts towards efficient overall water splitting. J Catal 2018;357:238-46.
48. Baek J, Hossain MD, Mukherjee P, et al. Synergistic effects of mixing and strain in high entropy spinel oxides for oxygen evolution reaction. Nat Commun 2023;14:5936.
49. Altaf A, Sohail M, Altaf M, Nafady A, Sher M, Wahab MA. Enhanced electrocatalytic activity of amorphized LaCoO3 for oxygen evolution reaction. Chem Asian J 2023:e202300870.
50. Smith RDL, Sporinova B, Fagan RD, Trudel S, Berlinguette CP. Facile photochemical preparation of amorphous iridium oxide films for water oxidation catalysis. Chem Mater 2014;26:1654-9.
51. Do VH, Prabhu P, Jose V, et al. Pd-PdO nanodomains on amorphous Ru metallene oxide for high-performance multifunctional electrocatalysis. Adv Mater 2023;35:e2208860.
52. Zhang L, Jang H, Liu H, et al. Sodium-decorated amorphous/crystalline RuO2 with rich oxygen vacancies: a robust pH-universal oxygen evolution electrocatalyst. Angew Chem Int Ed 2021;60:18821-9.
53. Li Y, Zhang X, Liu L, et al. Ultra-low Pt doping and Pt-Ni pair sites in amorphous/crystalline interfacial electrocatalyst enable efficient alkaline hydrogen evolution. Small 2023;19:e2300368.
54. Wang W, Shi X, He T, et al. Tailoring amorphous PdCu nanostructures for efficient C-C cleavage in ethanol electrooxidation. Nano Lett 2022;22:7028-33.
55. Jiang S, Zhu L, Yang Z, Wang Y. Self-supported hierarchical porous FeNiCo-based amorphous alloys as high-efficiency bifunctional electrocatalysts toward overall water splitting. Int J Hydrogen Energy 2021;46:36731-41.
56. Zhang X, Li L, Guo Y, Liu D, You T. Amorphous flower-like molybdenum-sulfide-@-nitrogen-doped-carbon-nanofiber film for use in the hydrogen-evolution reaction. J Colloid Interface Sci 2016;472:69-75.
57. Chen Z, Duan Z, Wang Z, et al. Amorphous cobalt oxide nanoparticles as active water-oxidation catalysts. ChemCatChem 2017;9:3641-5.
58. Pang Y, Xu W, Zhu S, et al. Self-supporting amorphous nanoporous NiFeCoP electrocatalyst for efficient overall water splitting. J Mater Sci Technol 2021;82:96-104.
59. Hu L, Zhao D, Liu C, et al. Amorphous CoB nanoarray as a high-efficiency electrocatalyst for nitrite reduction to ammonia. Inorg Chem Front 2022;9:6075-9.
60. Wang Z, You J, Zhao Y, et al. Research progress on high entropy alloys and high entropy derivatives as OER catalysts. J Environ Chem Eng 2023;11:109080.
61. Wang H, Wei R, Li X, Ma X, Hao X, Guan G. Nanostructured amorphous Fe29Co27Ni23Si9B12 high-entropy-alloy: an efficient electrocatalyst for oxygen evolution reaction. J Mater Sci Technol 2021;68:191-8.
62. Wang Q, Li J, Li Y, Shao G, Jia Z, Shen B. Non-noble metal-based amorphous high-entropy oxides as efficient and reliable electrocatalysts for oxygen evolution reaction. Nano Res 2022;15:8751-9.
63. Li M, Song M, Ni W, et al. Activating surface atoms of high entropy oxides for enhancing oxygen evolution reaction. Chin Chem Lett 2023;34:107571.
64. Yan G, Wang Y, Zhang Z, et al. Nanoparticle-decorated ultrathin La2O3 nanosheets as an efficient electrocatalysis for oxygen evolution reactions. Nanomicro Lett 2020;12:49.
65. Ghobrial S, Kirk DW, Thorpe SJ. Amorphous Ni-Nb-Y alloys as hydrogen evolution electrocatalysts. Electrocatalysis 2019;10:243-52.
66. Sun Y, Zhong S, Xin H, Zhang F, Chen L, Li X. Enhancement in oxidative property on amorphous rare earth doped Mn catalysts. Catal Commun 2016;77:94-7.
67. Gao J, Hou J, Kong L. Amorphous cobalt boride alloy synthesized by liquid phase methods as electrode materials for electrochemical capacitors. Part Part Syst Charact 2021;38:2100020.
68. Kong L, Dang S, Nie K, Han G, Tian G. Preparation of layered interconnected Si-Li2MnSiO4 electrode materials for the positive electrode of battery-type capacitors. Ionics 2022;28:5189-98.
69. Ye Y, Li K, Zhang W, Liu C. Precipitation of cesium lead halide perovskite nanocrystals in glasses based on liquid phase separation. J Am Ceram Soc 2022;105:6105-15.
70. Zhang H, Geng S, Ouyang M, Yadegari H, Xie F, Riley DJ. A self-reconstructed bifunctional electrocatalyst of pseudo-amorphous nickel carbide @ iron oxide network for seawater splitting. Adv Sci 2022;9:e2200146.
71. Wang A, Lin B, Zhang H, et al. Ambient temperature NO oxidation over Cr-based amorphous mixed oxide catalysts: effects from the second oxide components. Catal Sci Technol 2017;7:2362-70.
72. Liu W, Liu H, Dang L, et al. Amorphous cobalt-iron hydroxide nanosheet electrocatalyst for efficient electrochemical and photo-electrochemical oxygen evolution. Adv Funct Mater 2017;27:1603904.
73. Hasannaeimi V, Wang X, Salloom R, Xia Z, Schroers J, Mukherjee S. Nanomanufacturing of non-noble amorphous alloys for electrocatalysis. ACS Appl Energy Mater 2020;3:12099-107.
74. Balram A, Zhang H, Santhanagopalan S. Enhanced oxygen evolution reaction electrocatalysis via electrodeposited amorphous α-phase nickel-cobalt hydroxide nanodendrite forests. ACS Appl Mater Interfaces 2017;9:28355-65.
75. Wang W, Zhang K, Qiao Z, Li L, Liu P, Yang Y. Hydrodeoxygenation of p-cresol on unsupported Ni-W-Mo-S catalysts prepared by one step hydrothermal method. Catal Commun 2014;56:17-22.
76. Esquius J, Morgan DJ, Spanos I, Hewes DG, Freakley SJ, Hutchings GJ. Effect of base on the facile hydrothermal preparation of highly active IrOx oxygen evolution catalysts. ACS Appl Energy Mater 2020;3:800-9.
77. Chen G, Zhu Y, Chen HM, et al. An amorphous nickel-iron-based electrocatalyst with unusual local structures for ultrafast oxygen evolution reaction. Adv Mater 2019;31:e1900883.
78. Feng D, Wang P, Qin R, et al. Flower-like amorphous MoO3-x stabilized Ru single atoms for efficient overall water/seawater splitting. Adv Sci 2023;10:e2300342.
79. Zhong R, Liao Y, Peng L, et al. Silica-carbon nanocomposite acid catalyst with large mesopore interconnectivity by vapor-phase assisted hydrothermal treatment. ACS Sustain Chem Eng 2018;6:7859-70.
80. Karki S, Ingole PG. Development of polymer-based new high performance thin-film nanocomposite nanofiltration membranes by vapor phase interfacial polymerization for the removal of heavy metal ions. Chem Eng J 2022;446:137303.
81. Xia J, Li XZ, Huang X, et al. Physical vapor deposition synthesis of two-dimensional orthorhombic SnS flakes with strong angle/temperature-dependent Raman responses. Nanoscale 2016;8:2063-70.
82. Grüner C, Liedtke S, Bauer J, Mayr SG, Rauschenbach B. Morphology of thin films formed by oblique physical vapor deposition. ACS Appl Nano Mater 2018;1:1370-6.
83. Wei TR, Zhang SS, Liu Q, Qiu Y, Luo J, Liu X. Oxygen vacancy-rich amorphous copper oxide enables highly selective electroreduction of carbon dioxide to ethylene. Acta Physico Chimica Sinica 2022;2:20220702.
84. Hong YL, Liu Z, Wang L, et al. Chemical vapor deposition of layered two-dimensional MoSi2N4 materials. Science 2020;369:670-4.
85. Zhang ZJ, Sun HY, et al. Modulating p-d orbital hybridization by CuO/Cu nanoparticles enables carbon nanofibers high cycling stability as anode for sodium storage. Rare Met 2023;42:4039-47.
86. Su G, Hadjiev VG, Loya PE, et al. Chemical vapor deposition of thin crystals of layered semiconductor SnS2 for fast photodetection application. Nano Lett 2015;15:506-13.
87. Wu G, Zheng X, Cui P, et al. A general synthesis approach for amorphous noble metal nanosheets. Nat Commun 2019;10:4855.
88. Li K, Chen W. Recent progress in high-entropy alloys for catalysts: synthesis, applications, and prospects. Mater Today Energy 2021;20:100638.
89. Ricciardella F, Vollebregt S, Kurganova E, Giesbers AJM, Ahmadi M, Sarro PM. Growth of multi-layered graphene on molybdenum catalyst by solid phase reaction with amorphous carbon. 2D Mater 2019;6:035012.
90. Sundeev R, Shalimova A, Veligzhanin A, Glezer A, Zubavichus Y. Difference between local atomic structures of the amorphous
91. Yang X, Xu W, Cao S, et al. An amorphous nanoporous PdCuNi-S hybrid electrocatalyst for highly efficient hydrogen production. Appl Catal B Environ 2019;246:156-65.
92. Li H, Zhu Z, Wang Y, Chen S, Liu C, Zhang H. Disordered and oxygen vacancy-rich NiFe hydroxides/oxides in situ grown on amorphous ribbons for boosted alkaline water oxidation. J Electroanal Chem 2021;880:114918.
93. Wang ZJ, Li MX, Yu JH, Ge XB, Liu YH, Wang WH. Low-iridium-content IrNiTa metallic glass films as intrinsically active catalysts for hydrogen evolution reaction. Adv Mater 2020;32:e1906384.
94. Gou J, Qiao Z, Yu Z, et al. Architecting a 3D continuous C/CuVO3@Cu composite anode for lithium-ion storage. Surf Innov 2023;11:70-8.
95. Cole KM, Prabhudev S, Botton GA, Kirk DW, Thorpe SJ. Amorphous Ni-based nanoparticles for alkaline oxygen evolution. ACS Appl Nano Mater 2020;3:10522-30.
96. Duan Y, Yu ZY, Hu SJ, et al. Scaled-up synthesis of amorphous NiFeMo oxides and their rapid surface reconstruction for superior oxygen evolution catalysis. Angew Chem Int Ed 2019;58:15772-7.
97. Li Z, Xie Y, Huang Z, et al. Amorphization of LaCoO3 perovskite nanostructures for efficient oxygen evolution. ACS Appl Nano Mater 2022;5:14209-15.
98. Zhang M, Xu W, Ma CL, Yu J, Liu YT, Ding B. Highly active and selective electroreduction of N2 by the catalysis of Ga single atoms stabilized on amorphous TiO2 nanofibers. ACS Nano 2022;16:4186-96.
99. Lu J, Chen S, Zhuo Y, Mao X, Liu D, Wang Z. Greatly boosting seawater hydrogen evolution by surface amorphization and morphology engineering on MoO2/Ni3(PO4)2. Adv Funct Mater 2023;33:2308191.
100. Li X, Cai W, Li D, Xu J, Tao H, Liu B. Amorphous alloys for electrocatalysis: the significant role of the amorphous alloy structure. Nano Res 2023;16:4277-88.
101. Shen Y, Mai Y. Structural studies of amorphous and crystallized tungsten nitride thin films by EFED, XRD and TEM. Appl Surf Sci 2000;167:59-68.
102. Zhan C, Huang D, Hu X, et al. Mechanical property enhancement of NbTiZr refractory medium-entropy alloys due to Si-induced crystalline-to-amorphous transitions. Surf Coat Technol 2022;433:128144.
103. Dong C, Liu ZW, Liu JY, et al. Modest oxygen-defective amorphous manganese-based nanoparticle mullite with superior overall electrocatalytic performance for oxygen reduction reaction. Small 2017;13:1603903.
104. Pan T, Wang L, Shen Y, et al. Amorphous chromium oxide with hollow morphology for nitrogen electrochemical reduction under ambient conditions. ACS Appl Mater Interfaces 2022;14:14474-81.
105. Meng X, Yuan B, Liu Y, Zhao Z, Li K, Lin Y. Amorphous Fe-Mo-O nanostructures for catalytic water oxidation. ACS Appl Nano Mater 2022;5:9427-34.
106. Xu L, Tian Y, Deng D, et al. Cu nanoclusters/FeN4 amorphous composites with dual active sites in n-doped graphene for high-performance Zn-Air batteries. ACS Appl Mater Interfaces 2020;12:31340-50.
107. Chen C, Yan X, Wu Y, et al. Oxidation of metallic Cu by supercritical CO2 and control synthesis of amorphous nano-metal catalysts for CO2 electroreduction. Nat Commun 2023;14:1092.
108. Kang J, Yang X, Hu Q, Cai Z, Liu LM, Guo L. Recent progress of amorphous nanomaterials. Chem Rev 2023;123:8859-941.
109. Banko L, Krysiak OA, Pedersen JK, et al. Unravelling composition-activity-stability trends in high entropy alloy electrocatalysts by using a data-guided combinatorial synthesis strategy and computational modeling. Adv Energy Mater 2022;12:2103312.
110. Huang J, Fu C, Chen J, Senthilkumar N, Peng X, Wen Z. The enhancement of selectivity and activity for two-electron oxygen reduction reaction by tuned oxygen defects on amorphous hydroxide catalysts. CCS Chem 2022;4:566-83.
111. Fang Z, Shen B, Lu J, Fan K, Deng J. DFT study of electron transfer between B and Ni in Ni-B amorphous alloy. Acta Chimica Sinica 1999;57:894-900. Available from: https://sioc-journal.cn/Jwk_hxxb/EN/Y1999/V57/I8/894 [Last accessed on 9 Apr 2024].
112. He Y, Liu L, Zhu C, et al. Amorphizing noble metal chalcogenide catalysts at the single-layer limit towards hydrogen production. Nat Catal 2022;5:212-21.
113. Zhang D, Li H, Lu H, et al. Unlocking the performance of ternary metal (hydro)oxide amorphous catalysts via data-driven active-site engineering. Energy Environ Sci 2023;16:5065-75.
114. Bui TS, Lovell EC, Daiyan R, Amal R. Defective metal oxides: lessons from CO2 RR and applications in NOxRR. Adv Mater 2023;35:e2205814.
115. Ye L, Wang J, Zhang Y, Zhang M, Jing X, Gong Y. A self-supporting electrode with in-situ partial transformation of Fe-MOF into amorphous NiFe-LDH for efficient oxygen evolution reaction. Appl Surf Sci 2021;556:149781.
116. Zhao J, Ren X, Ma H, et al. Synthesis of self-supported amorphous CoMoO4 nanowire array for highly efficient hydrogen evolution reaction. ACS Sustain Chem Eng 2017;5:10093-8.
117. Yang P, Wang B, Liu Z. Towards activation of amorphous MoSx via Cobalt doping for enhanced electrocatalytic hydrogen evolution reaction. Int J Hydrogen Energy 2018;43:23109-17.
118. Wang D, Xie Y, Wu Z. Amorphous phosphorus-doped MoS2 catalyst for efficient hydrogen evolution reaction. Nanotechnology 2019;30:205401.
119. Ren H, Sun X, Du C, et al. Amorphous Fe-Ni-P-B-O nanocages as efficient electrocatalysts for oxygen evolution reaction. ACS Nano 2019;13:12969-79.
120. Dhandapani H, Madhu R, De A, Salem MA, Ramesh Babu B, Kundu S. Tuning the surface electronic structure of amorphous NiWO4 by doping Fe as an electrocatalyst for OER. Inorg Chem 2023;62:11817-28.
121. Sun J, Guo N, Shao Z, et al. A facile strategy to construct amorphous spinel-based electrocatalysts with massive oxygen vacancies using ionic liquid dopant. Adv Energy Mater 2018;8:1800980.
122. Gao YQ, Liu XY, Yang GW. Amorphous mixed-metal hydroxide nanostructures for advanced water oxidation catalysts. Nanoscale 2016;8:5015-23.
123. Yang M, Zhao M, Yuan J, et al. Oxygen vacancies and interface engineering on amorphous/crystalline CrOx-Ni3 N heterostructures toward high-durability and kinetically accelerated water splitting. Small 2022;18:e2106554.
124. Gao L, Guo C, Sun X, et al. CoFeOx(OH)y/CoOx(OH)y core/shell structure with amorphous interface as an advanced catalyst for electrocatalytic water splitting. Electrochim Acta 2020;341:136038.
125. Sun A, Qiu Y, Wang Z, et al. Interface engineering on super-hydrophilic amorphous/crystalline NiFe-based hydroxide/selenide heterostructure nanoflowers for accelerated industrial overall water splitting at high current density. J Colloid Interface Sci 2023;650:573-81.
126. Zhang HM, Zuo LH, Gao YH, et al. Amorphous high-entropy phosphoxides for efficient overall alkaline water/seawater splitting. J Mater Sci Technol 2023;173:1-10.
127. Cheng C, Liu T, Wang Y, et al. Amorphous Sn(HPO4)2-derived phosphorus-modified Sn/SnO core/shell catalyst for efficient CO2 electroreduction to formate. J Energy Chem 2023;81:125-31.
128. Smith G, Brower W, Matyjaszczyk M, Pettit T. Metallic glasses: new catalyst systems. Stud Surf Sci Catal 1981;7:355-63.
129. Kreysa G, Håkansson B. Electrocatalysis by amorphous metals of hydrogen and oxygen evolution in alkaline solution. J Electroanal Chem Interfacial Electrochem 1986;201:61-83.
130. Lian K, Thorpe S, Kirk D. Electrochemical and surface characterization of electrocatalytically active amorphous Ni Co alloys. Electrochim Acta 1992;37:2029-41.
131. Deng J, Li H, Wang W. Progress in design of new amorphous alloy catalysts. Catal Today 1999;51:113-25.
132. Janik-czachor M, Szummer A, Molnar A, et al. Electrochemical modification of Cu-Zr amorphous alloys for catalysts. Electrochim Acta 2000;45:3295-304.
133. Li H, Xu Y. Liquid phase benzene hydrogenation to cyclohexane over modified Ni-P amorphous catalysts. Mater Lett 2001;51:101-7.
134. Ramos-sánchez G, Pierna A, Solorza-feria O. Amorphous Ni59Nb40PtxM1-x (M=Ru,Sn) electrocatalysts for oxygen reduction reaction. J Non-Cryst Solids 2008;354:5165-8.
135. Fernandes R, Patel N, Miotello A. Efficient catalytic properties of Co-Ni-P-B catalyst powders for hydrogen generation by hydrolysis of alkaline solution of NaBH4. Int J Hydrogen Energy 2009;34:2893-900.
136. Fugane K, Mori T, Ou DR, et al. Activity of oxygen reduction reaction on small amount of amorphous CeOx promoted Pt cathode for fuel cell application. Electrochim Acta 2011;56:3874-83.
137. Cavalca F, Ferragut R, Aghion S, et al. Nature and distribution of stable subsurface oxygen in copper electrodes during electrochemical CO2 reduction. J Phys Chem C 2017;121:25003-9.
138. Lv CD, Yan CS, Chen G, et al. An amorphous noble-metal-free electrocatalyst that enables nitrogen fixation under ambient conditions. Angew Chem Int Ed 2018;130:6181-4.
139. Tielens F, Gierada M, Handzlik J, Calatayud M. Characterization of amorphous silica based catalysts using DFT computational methods. Catal Today 2020;354:3-18.
140. Liu H, Xi C, Xin J, et al. Free-standing nanoporous NiMnFeMo alloy: an efficient non-precious metal electrocatalyst for water splitting. Chem Eng J 2021;404:126530.
141. Pu Z, Amiinu IS, Cheng R, et al. Single-atom catalysts for electrochemical hydrogen evolution reaction: recent advances and future perspectives. Nanomicro Lett 2020;12:21.
142. Zheng X, Li P, Dou S, et al. Non-carbon-supported single-atom site catalysts for electrocatalysis. Energy Environ Sci 2021;14:2809-58.
143. Al-naggar AH, Shinde NM, Kim J, Mane RS. Water splitting performance of metal and non-metal-doped transition metal oxide electrocatalysts. Coord Chem Rev 2023;474:214864.
144. Xing M, Zhu S, Zeng X, Wang S, Liu Z, Cao D. Amorphous/Crystalline Rh(OH)3/CoP heterostructure with hydrophilicity/aerophobicity feature for all-pH hydrogen evolution reactions. Adv Energy Mater 2023;13:2302376.
145. Cao D, Dong Y, Tang Y, et al. Amorphous manganese-cobalt nanosheets as efficient catalysts for hydrogen evolution reaction (HER). Catal Surv Asia 2021;25:437-44.
146. Xia Y, Wu W, Wang H, Rao S, Zhang F, Zou G. Amorphous RuS2 electrocatalyst with optimized active sites for hydrogen evolution. Nanotechnology 2020;31:145401.
147. Zhang X, Li K, Wen B, Ma J, Diao D. Machine learning accelerated DFT research on platinum-modified amorphous alloy surface catalysts. Chin Chem Lett 2023;34:107833.
148. Zhang J, Wu Y, Hao H, et al. Construction of amorphous Fe0.95S1.05 nanorods with high electrocatalytic activity for enhanced hydrogen evolution reaction. Electrochim Acta 2022;402:139554.
149. Wu G, Han X, Cai J, et al. In-plane strain engineering in ultrathin noble metal nanosheets boosts the intrinsic electrocatalytic hydrogen evolution activity. Nat Commun 2022;13:4200.
150. Chunduri A, Bhide A, Gupta S, et al. Exploring the role of multi-catalytic sites in an amorphous Co-W-B electrocatalyst for hydrogen and oxygen evolution reactions. ACS Appl Energy Mater 2023;6:4630-41.
151. Shao G, Wang Q, Miao F, Li J, Li Y, Shen B. Improved catalytic efficiency and stability by surface activation in Fe-based amorphous alloys for hydrogen evolution reaction in acidic electrolyte. Electrochim Acta 2021;390:138815.
152. Yu J, Li A, Li L, Li X, Wang X, Guo L. Morphological and structural engineering in amorphous Cu2MoS4 nanocages for remarkable electrocatalytic hydrogen evolution. Sci China Mater 2019;62:1275-84.
153. Zhao L, Chang B, Dong T, et al. Laser synthesis of amorphous CoSx nanospheres for efficient hydrogen evolution and nitrogen reduction reactions. J Mater Chem A 2022;10:20071-9.
154. Lu W, Li X, Wei F, et al. In-situ transformed Ni, S-Codoped CoO from amorphous Co-Ni sulfide as an efficient electrocatalyst for hydrogen evolution in alkaline media. ACS Sustain Chem Eng 2019;7:12501-9.
155. Hu M, Qian Y, Yu S, et al. Amorphous MoS2 decorated Ni3S2 with a core-shell structure of urchin-like on nickel-foam efficient hydrogen evolution in acidic and alkaline media. Small 2024;20:e2305948.
156. Ge Y, Ge J, Huang B, et al. Synthesis of amorphous Pd-based nanocatalysts for efficient alcoholysis of styrene oxide and electrochemical hydrogen evolution. Nano Res 2023;16:4650-5.
157. Bodhankar PM, Sarawade PB, Kumar P, et al. Nanostructured metal phosphide based catalysts for electrochemical water splitting: a review. Small 2022;18:e2107572.
158. Jamesh M, Sun X. Recent progress on earth abundant electrocatalysts for oxygen evolution reaction (OER) in alkaline medium to achieve efficient water splitting - a review. J Power Sources 2018;400:31-68.
159. Li X, Zhang H, Hu Q, et al. Amorphous NiFe oxide-based nanoreactors for efficient electrocatalytic water oxidation. Angew Chem Int Ed 2023;62:e202300478.
160. Liu S, Geng S, Li L, et al. A top-down strategy for amorphization of hydroxyl compounds for electrocatalytic oxygen evolution. Nat Commun 2022;13:1187.
161. Liu X, Yin H, Zhang S, et al. Revealing the effect of crystallinity and oxygen vacancies of Fe-Co phosphate on oxygen evolution for high-current water splitting. J Colloid Interface Sci 2024;653:1379-87.
162. Xiao L, Liang Y, Li Z, et al. Amorphous FeNiNbPC nanoprous structure for efficient and stable electrochemical oxygen evolution. J Colloid Interface Sci 2022;608:1973-82.
163. Zheng Y, Guo R, Li X, et al. Synthesis of amorphous trimetallic PdCuNiP nanoparticles for enhanced OER. Front Chem 2023;11:1122333.
164. Zhao C, Li N, Zhang R, et al. Surface reconstruction of La0.8Sr0.2Co0.8Fe0.2O3-δ for superimposed OER performance. ACS Appl Mater Interfaces 2019;11:47858-67.
165. Kalathil S, Katuri KP, Saikaly PE. Synthesis of an amorphous Geobacter -manganese oxide biohybrid as an efficient water oxidation catalyst. Green Chem 2020;22:5610-8.
166. Gou W, Xia Z, Tan X, et al. Highly active and stable amorphous IrOx/CeO2 nanowires for acidic oxygen evolution. Nano Energy 2022;104:107960.
167. Zhang L, Lu C, Ye F, et al. Vacancies boosting strategy enabling enhanced oxygen evolution activity in a library of novel amorphous selenite electrocatalysts. Appl Catal B Environ 2021;284:119758.
168. Wang S, Zhao R, Zheng T, et al. Cerium decorated amorphous ternary Ni-Ce-B catalyst for enhanced electrocatalytic water oxidation. Surf Interfaces 2021;26:101447.
169. Li Z, Li C, Huang J, et al. Structure engineering of amorphous P-CoS hollow electrocatalysts for promoted oxygen evolution reaction. Int J Hydrogen Energy 2022;47:15189-97.
170. Kim C, Dionigi F, Beermann V, Wang X, Möller T, Strasser P. Alloy nanocatalysts for the electrochemical oxygen reduction (ORR) and the direct electrochemical carbon dioxide reduction reaction (CO2RR). Adv Mater 2019;31:e1805617.
171. Tian J, Shen Y, Liu P, et al. Recent advances of amorphous-phase-engineered metal-based catalysts for boosted electrocatalysis. J Mater Sci Technol 2022;127:1-18.
172. Biemolt J, Rothenberg G, Yan N. Understanding the roles of amorphous domains and oxygen-containing groups of nitrogen-doped carbon in oxygen reduction catalysis: toward superior activity. Inorg Chem Front 2020;7:177-85.
173. Liu L, Zhao X, Li R, Su H, Zhang H, Liu Q. Subnano amorphous Fe-based clusters with high mass activity for efficient electrocatalytic oxygen reduction reaction. ACS Appl Mater Interfaces 2019;11:41432-9.
174. Poon KC, Tan DC, Vo TD, et al. Newly developed stepwise electroless deposition enables a remarkably facile synthesis of highly active and stable amorphous Pd nanoparticle electrocatalysts for oxygen reduction reaction. J Am Chem Soc 2014;136:5217-20.
175. Bai F, He Y, Xu L, et al. Improved ORR/OER bifunctional catalytic performance of amorphous manganese oxides prepared by photochemical metal-organic deposition. RSC Adv 2022;12:2408-15.
176. Wang Y, Liu J, Yuan H, Liu F, Hu T, Yang B. Strong electronic interaction between amorphous MnO2 nanosheets and ultrafine Pd nanoparticles toward enhanced oxygen reduction and ethylene glycol oxidation reactions. Adv Funct Mater 2023;33:2211909.
177. Li W, Fu W, Bai S, et al. Inspired electrocatalytic performance by unique amorphous PdCu nanoparticles on black phosphorus. Electrochim Acta 2023;446:142082.
178. Li Q, Kong D, Zhao X, et al. Short-range amorphous carbon nanosheets for oxygen reduction electrocatalysis. Nanoscale Adv 2020;2:5769-76.
179. Pan D, Chen P, Zhou L, Liu J, Guo Z, Song J. Self-template construction of 2D amorphous N-doped CoFe-mesoporous phosphate microsheets for zinc-air batteries. J Power Sources 2021;498:229859.
180. Song S, Li W, Deng Y, et al. TiC supported amorphous MnOx as highly efficient bifunctional electrocatalyst for corrosion resistant oxygen electrode of Zn-air batteries. Nano Energy 2020;67:104208.
181. Moloudi M, Noori A, Rahmanifar MS, et al. Layered double hydroxide templated synthesis of amorphous NiCoFeB as a multifunctional electrocatalyst for overall water splitting and rechargeable zinc-air batteries. Adv Energy Mater 2023;13:2203002.
182. Jin D, Lee Y, Kim IY, Lee C, Kim MH. Impact of controlling the crystallinity on bifunctional electrocatalytic performances toward methanol oxidation and oxygen reduction in binary Pd-Cr solid solution. J Mater Chem A 2023;11:16243-54.
183. Yao Y, Zhuang W, Li R, et al. Sn-based electrocatalysts for electrochemical CO2 reduction. Chem Commun 2023;59:9017-28.
184. Han H, Jin S, Park S, et al. Plasma-induced oxygen vacancies in amorphous MnOx boost catalytic performance for electrochemical CO2 reduction. Nano Energy 2021;79:105492.
185. Xiong Y, Wei B, Wu M, et al. Rapid synthesis of amorphous bimetallic copper-bismuth electrocatalysts for efficient electrochemical CO2 reduction to formate in a wide potential window. J CO2 Util 2021;51:101621.
186. Duan YX, Meng FL, Liu KH, et al. Amorphizing of Cu nanoparticles toward highly efficient and robust electrocatalyst for CO2 reduction to liquid fuels with high faradaic efficiencies. Adv Mater 2018;30:e1706194.
187. Zhang J, Yin R, Shao Q, Zhu T, Huang X. Oxygen vacancies in amorphous InOx nanoribbons enhance CO2 adsorption and activation for CO2 electroreduction. Angew Chem Int Ed 2019;58:5609-13.
188. Wu Y, Zhai P, Cao S, et al. Beyond d orbits: steering the selectivity of electrochemical CO2 reduction via hybridized sp band of sulfur-incorporated porous Cd architectures with dual collaborative sites. Adv Energy Mater 2020;10:2002499.
189. Zhou JH, Yuan K, Zhou L, et al. Boosting electrochemical reduction of CO2 at a low overpotential by amorphous Ag-Bi-S-O decorated Bi0 nanocrystals. Angew Chem Int Ed 2019;58:14197-201.
190. Dong Z, Sun Q, Xu GR, et al. Universal synthesized strategy for amorphous Pd-Based nanosheets boosting ambient ammonia electrosynthesis. Small Methods 2023;7:e2201225.
191. Xu W, Zhang M, Ma C, Wu S, Liu Y. Amorphous NiSb2O6-x nanofiber: a d-/p-block Janus electrocatalyst toward efficient NH3 synthesis through boosted N2 adsorption and activation. Appl Catal B Environ 2022;308:121225.
192. Wang Y, Tian Y, Zhang J, et al. Tuning morphology and electronic structure of amorphous NiFeB nanosheets for enhanced electrocatalytic N2 reduction. ACS Appl Energy Mater 2020;3:9516-22.
193. Chu K, Nan H, Li Q, Guo Y, Tian Y, Liu W. Amorphous MoS3 enriched with sulfur vacancies for efficient electrocatalytic nitrogen reduction. J Energy Chem 2021;53:132-8.
194. Xiao L, Liang Y, Li Z, et al. Amorphous CoMoO4 with nanoporous structures for electrochemical ammonia synthesis under ambient conditions. ACS Sustain Chem Eng 2020;8:19072-83.
Cite This Article
Export citation file: BibTeX | RIS
OAE Style
Yu Z, Sun Q, Zhang L, Yang H, Chen Y, Guo J, Zhang M, Zhang Z, Jiang Y. Research progress of amorphous catalysts in the field of electrocatalysis. Microstructures 2024;4:2024022. http://dx.doi.org/10.20517/microstructures.2023.66
AMA Style
Yu Z, Sun Q, Zhang L, Yang H, Chen Y, Guo J, Zhang M, Zhang Z, Jiang Y. Research progress of amorphous catalysts in the field of electrocatalysis. Microstructures. 2024; 4(2): 2024022. http://dx.doi.org/10.20517/microstructures.2023.66
Chicago/Turabian Style
Yu, Zhenyang, Qi Sun, Lianwang Zhang, Huan Yang, Yuefang Chen, Junpeng Guo, Mengmeng Zhang, Zhijia Zhang, Yong Jiang. 2024. "Research progress of amorphous catalysts in the field of electrocatalysis" Microstructures. 4, no.2: 2024022. http://dx.doi.org/10.20517/microstructures.2023.66
ACS Style
Yu, Z.; Sun Q.; Zhang L.; Yang H.; Chen Y.; Guo J.; Zhang M.; Zhang Z.; Jiang Y. Research progress of amorphous catalysts in the field of electrocatalysis. Microstructures. 2024, 4, 2024022. http://dx.doi.org/10.20517/microstructures.2023.66
About This Article
Special Issue
Copyright
Data & Comments
Data
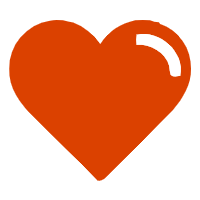

Comments
Comments must be written in English. Spam, offensive content, impersonation, and private information will not be permitted. If any comment is reported and identified as inappropriate content by OAE staff, the comment will be removed without notice. If you have any queries or need any help, please contact us at support@oaepublish.com.