Arginine modification of hybrid cobalt/nitrogen Ti3C2Tx MXene and its application as a sulfur host for lithium-sulfur batteries
Abstract
The shuttling effect of lithium polysulfides (LiPSs) is one of the challenges facing the commercialization, which leads to a significant capacity degradation. This paper proposes a novel method to promote polysulfide transformation by employing arginine to regulate the layer spacing of cobalt-nitrogen doped
Keywords
INTRODUCTION
Lightweight and long service rechargeable batteries have been developed to satisfy the increasing demands of industrial needs, military progress and portable life[1-3]. Furthermore, the development of advanced electrochemical energy conversion and storage systems with a high energy density has been gaining significant attention[4-7]. Despite the various advantages of existing lithium-ion batteries, their low energy density based on the intercalation mechanism has severely hindered the scale-up fabrication of miniature batteries[5]. Lithium-sulfur (Li-S) batteries with high theoretical specific capacity (1,675 mAh g-1) and energy density (2,567 Wh kg-1) have attracted considerable attention as the next-generation batteries[8-11]. However, their low sulfur utilization, sluggish redox reaction kinetics, fast capacity fading, and low coulombic efficiency (CE) have hindered their commercialization[12,13].
To address these issues, several studies have reported the development of advanced sulfur host materials for the Li-S cathodes[14,15]. For example, carbon materials and conducting material with large specific surface area have been used to inhibit the shuttle effect of polysulfide. In particular, two-dimensional conductive carbon materials have been employed owing to their adjustable morphology and high electrical conductivity[16-18]. Nevertheless, the interactions are weak between polar lithium polysulfides (LiPSs) and the nonpolar hydrophobic carbon framework play a very limited role in slowing down the shuttle effect or preventing interfacial charge transfer, thus leading to sluggish reaction kinetics[17,19,20].
The application of
Several strategies have focused on increasing the layer spacing and modifying the
This paper reports the fabrication of amino acid-regulated Co, N-doped
MATERIALS AND METHODS
Materials
400 mesh powder of Ti3AlC2 was from XF Nano Technology Co., Ltd. (Nanjing, China), while serine, lysine, arginine, LiF, 4-dimethylaminopyridine (DMAP) and 1-(3-(dimethylamino) propyl)-3-ethylcarbimide hydrochloride (EDC), cobalt chloride hexahydrate were purchased from Titan Scientific Co., Ltd. (General-Reagent brand). Concentrated HCl (36.5%) was obtained from Sinopharm Chemical Reagent Co., Ltd.
Preparation of the Ti3C2Tx-Arg, Ti3C2Tx-Ser, Ti3C2Tx-Lys composites
The
Preparation of the Co-N@Ti3C2Tx-Arg @S cathode
Each of the Ti3C2TX-Arg, Ti3C2TX-Ser, and Ti3C2TX-Lys film was immersed in a CoCl2 solution (0.1 mol·L-1) for 2 h. After drying, each film was then annealed at 600 °C under an Ar atmosphere for 4 h (temperature rise of
The S composite cathode was prepared as follows
The
Characterization
The morphologies were imaged by a scanning electron microscope (SEM, ZEISS Sigma 300) at an increased voltage of 3 kV equipped with an energy-dispersive spectrometer (EDS, Oxford Xplore50, pure gold target with an accelerated voltage of 0.02 to 30 kV). The X-ray diffraction (XRD) patterns were recorded on a Rigaku Smart Lab (Japan) equipped with a CuKα light source (λ = 0.15406 nm). Samples were scanned in the diffraction angle (2θ) range of 5° to 60° at a scanning rate of 2°/min. The X-ray photoelectron spectroscopy (XPS) spectra were recorded on a Thermo Scientific K-Alpha model spectrometer. The entire spectral scan was performed with a universal energy of 100 eV and a step size of 1 eV. The Thermo Fisher DXR 2xi model Raman imaging microscope with a spectral resolution of less than 1.5 cm-1 was used for the Raman spectroscopy analysis.
Electrochemical measurements
The mass of sulfur in the electrode was in the range of 1.0-1.5 mg. The discs were then tested with CR2032 coin-type cells using lithium metal as the anode, Celgard 2400 as the separator, and the electrolyte solution was composed of a LiTFSI (1 M) dissolved in a solution of 1,3-dioxolane (DOL) and dimethoxymethane (DME) (v:v = 1:1) solution containing LiNO3 (1 wt.%) as the additive. The Charge and discharge measurements were conducted in the potential range of 1.5-3.0 V (vs. Li/Li+) on a LAND testing system. electrolyte amount was 20 µL mg-1. The cyclic voltammetry (CV) and electrochemical impedance spectroscopy (EIS) were conducted using DE7000 potentiostat/galvanostat in the frequency range of 10 kHz to 0.1 Hz.
RESULTS AND DISCUSSION
Figure 1 illustrates the procedure for the synthesis of the Co-N@Ti3C2Tx-Ser, Co-N@Ti3C2Tx-Lys, and
The SEM results of the Ti3AlC2 and etched multilayer
Figure 2. SEM images of the (A and B) multilayer Ti3C2TX, (C-E) Ti3C2TX-Ser, and Ti3C2TX-Lys, and Ti3C2Tx-Arg, composites; (F-J) SEM image and EDS spectrum mapping: C, Co, and N elemental distribution of the
The schematic representation of the amino-acid-modified
Figure 3. (A) Schematic illustration of the
The possibility to employ each of the obtained composites as an S cathode was then investigated [Figure 4]. Free-standing
Figure 4. (A) Schematic illustration of the fabrication of the Co-N@Ti3C2TX-Arg/S electrodes for Li-S batteries. (B) Rate performances of the Co-N@Ti3C2TX-Ser/S, Co-N@Ti3C2TX-Lys /S, and Co-N@Ti3C2TX-Arg/S electrodes. (C) Long-term cycling of the Co-N@Ti3C2TX-Ser/S, Co-N@Ti3C2TX-Lys/S, and Co-N@Ti3C2TX-Arg/S electrodes. (D) CEs of the Co-N@Ti3C2TX-Ser/S, Co-N@Ti3C2TX-Lys /S and
CONCLUSIONS
This study reported the synthesis of Co, N doped
DECLARATIONS
Authors’ contributions
Synthesis and testing of materials, data collection, original manuscript writing: Zhang M
Validation and original manuscript revision: Zhang K
Data analysis: Wei W
manuscript Revision: Yuan H
Reviewing and editing: Chang J
Revision: Hao Y
Availability of data and materials
According to reasonable requirements, all of the data examined in this research can be obtained from the correspondents.
Financial support and sponsorship
This work was financially supported by the National Key Research and Development Program of China (Grants 2021YFA0715600, 2021YFA0717700), National Natural Science Foundation of China (52192610, 62274127, 62374128), Youth Project of Natural Science Basic Research Program of Shaanxi Province (2021JQ-189), Fundamental Research Funds for the Central Universities, and Innovation Fund of Xidian University.
Conflicts of interest
All authors declared that there are no conflicts of interest.
Ethical approval and consent to participate
Not applicable.
Consent for publication
Not applicable.
Copyright
© The Author(s) 2024.
Supplementary Materials
REFERENCES
1. Chang P, Mei H, Zhou S, Dassios KG, Cheng L. 3D printed electrochemical energy storage devices. J Mater Chem A 2019;7:4230-58.
2. Xia J, Gao R, Yang Y, et al. TinO2n-1/MXene hierarchical bifunctional catalyst anchored on graphene aerogel toward flexible and high-energy Li-S batteries. ACS Nano 2022;16:19133-44.
3. Liu K, Fan Y, Ali A, Shen PK. A flexible and conductive MXene-coated fabric integrated with in situ sulfur loaded MXene nanosheets for long-life rechargeable Li-S batteries. Nanoscale 2021;13:2963-71.
4. Chang P, Mei H, Zhao Y, Huang W, Zhou S, Cheng L. 3D structural strengthening urchin-like Cu(OH)2-based symmetric supercapacitors with adjustable capacitance. Adv Funct Mater 2019;29:1903588.
5. Chang P, Mei H, Zhang M, et al. 3D printed electrochromic supercapacitors with ultrahigh mechanical strength and energy density. Small 2021;17:e2102639.
6. Song MK, Zhang Y, Cairns EJ. A long-life, high-rate lithium/sulfur cell: a multifaceted approach to enhancing cell performance. Nano Lett 2013;13:5891-9.
7. Xu T, Du H, Liu H, et al. Advanced nanocellulose-based composites for flexible functional energy storage devices. Adv Mater 2021;33:e2101368.
8. Zhong X, Wang D, Sheng J, et al. Freestanding and sandwich MXene-based cathode with suppressed lithium polysulfides shuttle for flexible lithium-sulfur batteries. Nano Lett 2022;22:1207-16.
9. Peng HJ, Zhang G, Chen X, et al. Enhanced electrochemical kinetics on conductive polar mediators for lithium-sulfur batteries. Angew Chem Int Ed 2016;55:12990-5.
10. Huang Z, Zhu Y, Kong Y, et al. Efficient synergism of chemisorption and wackenroder reaction via heterostructured La2O3-Ti3C2Tx-embedded carbon nanofiber for high-energy lithium-sulfur pouch cells. Adv Funct Mater 2023;33:2303422.
11. Nam S, Kim J, Nguyen VH, et al. Collectively exhaustive MXene and graphene oxide multilayer for suppressing shuttling effect in flexible lithium sulfur battery. Adv Mater Technol 2022;7:2101025.
12. Li H, Shao F, Wen X, et al. Graphene/MXene fibers-enveloped sulfur cathodes for high-performance Li-S batteries. Electrochim Acta 2021;371:137838.
13. Qiu Y, Li W, Zhao W, et al. High-rate, ultralong cycle-life lithium/sulfur batteries enabled by nitrogen-doped graphene. Nano Lett 2014;14:4821-7.
14. Hu B, Xu J, Fan Z, et al. Covalent organic framework based lithium-sulfur batteries: materials, interfaces, and solid-state electrolytes. Adv Energy Mater 2023;13:2203540.
15. Zheng Z, Ye H, Guo Z. Recent progress on pristine metal/covalent-organic frameworks and their composites for lithium-sulfur batteries. Energy Environ Sci 2021;14:1835-53.
16. Zheng X, Tang J, Wang P, Wang Z, Zou L, Li C. Interfused core-shell heterogeneous graphene/MXene fiber aerogel for high-performance and durable electromagnetic interference shielding. J Colloid Interface Sci 2022;628:994-1003.
17. Liu Y, He P, Zhou H. Rechargeable solid-state Li-air and Li-S batteries: materials, construction, and challenges. Adv Energy Mater 2018;8:1701602.
18. Li Z, Huang Y, Yuan L, Hao Z, Huang Y. Status and prospects in sulfur-carbon composites as cathode materials for rechargeable lithium-sulfur batteries. Carbon 2015;92:41-63.
19. Zhao Q, Zhu Q, Liu Y, Xu B. Status and prospects of MXene-based lithium-sulfur batteries. Adv Funct Mater 2021;31:2100457.
20. Feng Y, Liu H, Lu Q. From non-carbon host toward carbon-free lithium-sulfur batteries. Nano Res 2023;17:1337-65.
21. Chang P, Mei H, Zhao Y, et al. Nature-inspired 3D spiral grass structured graphene quantum dots/MXene nanohybrids with exceptional photothermal-driven pseudo-capacitance improvement. Adv Sci 2022;9:e2204086.
22. Zhang Y, Ma C, He W, et al. MXene and MXene-based materials for lithium-sulfur batteries. Prog Nat Sci Mater 2021;31:501-13.
23. Dong Y, Zheng S, Qin J, et al. All-MXene-based integrated electrode constructed by Ti3C2 nanoribbon framework host and nanosheet interlayer for high-energy-density Li-S batteries. ACS Nano 2018;12:2381-8.
24. Tang H, Li W, Pan L, et al. A robust, freestanding MXene-sulfur conductive paper for long-lifetime Li-S batteries. Adv Funct Mater 2019;29:1901907.
25. Wu Z, Liu X, Shang T, et al. Reassembly of MXene hydrogels into flexible films towards compact and ultrafast supercapacitors. Adv Funct Mater 2021;31:2102874.
26. Xiao Z, Li Z, Li P, Meng X, Wang R. Ultrafine Ti3C2 MXene nanodots-interspersed nanosheet for high-energy-density lithium-sulfur batteries. ACS Nano 2019;13:3608-17.
27. Zhang Y, Mu Z, Yang C, et al. Rational design of MXene/1T-2H MoS2-C nanohybrids for high-performance lithium-sulfur batteries. Adv Funct Mater 2018;28:1707578.
28. Bao W, Liu L, Wang C, Choi S, Wang D, Wang G. Facile Synthesis of crumpled nitrogen-doped MXene nanosheets as a new sulfur host for lithium-sulfur batteries. Adv Energy Mater 2018;8:1702485.
29. Zhang H, Yang L, Zhang P, et al. MXene-Derived TinO2n-1 quantum dots distributed on porous carbon nanosheets for stable and long-life Li-S batteries: enhanced polysulfide mediation via defect engineering. Adv Mater 2021;33:e2008447.
30. Tian S, Huang J, Yang H, et al. Self-supporting multicomponent hierarchical network aerogel as sulfur anchoring-catalytic medium for highly stable lithium-sulfur battery. Small 2022;18:e2205163.
31. Zhang C, Cui L, Abdolhosseinzadeh S, Heier J. Two-dimensional MXenes for lithium-sulfur batteries. InfoMat 2020;2:613-38.
32. Zou J, Wu J, Wang Y, et al. Additive-mediated intercalation and surface modification of MXenes. Chem Soc Rev 2022;51:2972-90.
33. Bae J, Qian Y, Li Y, Zhou X, Goodenough JB, Yu G. Polar polymer-solvent interaction derived favorable interphase for stable lithium metal batteries. Energy Environ Sci 2019;12:3319-27.
34. Fan M, Cui J, Wu J, Vajtai R, Sun D, Ajayan PM. Improving the catalytic activity of carbon-supported single atom catalysts by polynary metal or heteroatom doping. Small 2020;16:e1906782.
35. Liu S, Li J, Yan X, et al. Superhierarchical cobalt-embedded nitrogen-doped porous carbon nanosheets as two-in-one hosts for high-performance lithium-sulfur batteries. Adv Mater 2018;30:e1706895.
36. Naguib M, Kurtoglu M, Presser V, et al. Two-dimensional nanocrystals produced by exfoliation of Ti3AlC2. Adv Mater 2011;23:4248-53.
37. Guo Q, Zhang X, Zhao F, et al. Protein-inspired self-healable Ti3C2 MXenes/rubber-based supramolecular elastomer for intelligent sensing. ACS Nano 2020;14:2788-97.
38. Peng M, Wang L, Li L, et al. Manipulating the interlayer spacing of 3D MXenes with improved stability and zinc-ion storage capability. Adv Funct Mater 2022;32:2109524.
39. Tan Y, Xu C, Chen G, et al. Synthesis of ultrathin nitrogen-doped graphitic carbon nanocages as advanced electrode materials for supercapacitor. ACS Appl Mater Interfaces 2013;5:2241-8.
40. Chen Y, Li Z, Zhu Y, et al. Atomic Fe dispersed on N-doped carbon hollow nanospheres for high-efficiency electrocatalytic oxygen reduction. Adv Mater 2019;31:e1806312.
Cite This Article
Export citation file: BibTeX | RIS
OAE Style
Zhang M, Zhang K, Wei W, Yuan H, Chang J, Hao Y. Arginine modification of hybrid cobalt/nitrogen
AMA Style
Zhang M, Zhang K, Wei W, Yuan H, Chang J, Hao Y. Arginine modification of hybrid cobalt/nitrogen
Chicago/Turabian Style
Zhang, Miao, Kaiyu Zhang, Wei Wei, Hongxin Yuan, Jingjing Chang, Yue Hao. 2024. "Arginine modification of hybrid cobalt/nitrogen
ACS Style
Zhang, M.; Zhang K.; Wei W.; Yuan H.; Chang J.; Hao Y. Arginine modification of hybrid cobalt/nitrogen
About This Article
Special Issue
Copyright
Data & Comments
Data
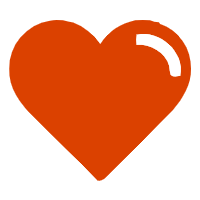

Comments
Comments must be written in English. Spam, offensive content, impersonation, and private information will not be permitted. If any comment is reported and identified as inappropriate content by OAE staff, the comment will be removed without notice. If you have any queries or need any help, please contact us at support@oaepublish.com.