Hierarchically structured biomaterials for tissue regeneration
Abstract
Repairing tissue defects caused by diseases and traumas presents significant challenges in the clinic. Recent advancements in biomaterials have offered promising strategies for promoting tissue regeneration. In particular, the exploration of 3D macro and microstructures of biomaterials has proven crucial in this process. The integration of macro, micro, and nanostructures facilitates the performance of biomaterials in terms of their mechanical properties, degradation rate, and distinctive impacts on cellular activities. In this review, we summarize the recent progress in biomaterials with hierarchical structures for tissue regeneration. We explore the various methods and strategies employed in designing biomaterials with hierarchical structures of different dimensions. The improvement of physicochemical properties and bioactivities by hierarchically structured biomaterials, including the regulation of mechanical properties, degradability, and the specific functions of cell behaviors, has been highlighted. Furthermore, the current applications of hierarchically structured biomaterials for tissue regeneration are discussed. Finally, we conclude by summarizing the developments of hierarchically structured biomaterials for tissue regeneration and provide future perspectives.
Keywords
INTRODUCTION
Tissue defects resulting from accidents, aging, cancer, and other diseases have profoundly affected individuals’ lives. Regrettably, the clinic is confronted with significant challenges when achieving rapid and effective tissue regeneration[1]. It is worth noting that tissue engineering offers the remarkable potential for efficient and prompt tissue regeneration[2]. As a critical part of tissue engineering, the design and development of biomaterials with different structures and bioactivities are particularly crucial. Biomaterials with diverse structures have been applied for tissue regeneration, providing essential physicochemical cues and bioactivities[3]. Reasonable composition design of biomaterials can provide bioactive clues for tissue defects; for example, bioactive ions released (Si, Mg, Mn, Fe, Ca, Sr, etc.) from biomaterials are beneficial for the improvement of cell activities and regulation of microenvironment, finally promoting tissue regeneration[4]. Moreover, tissue regeneration caused by diseases, such as tumors and trauma, usually needs biomaterials that possess additional therapeutic functions, such as antibacterial and antitumor properties.
Generally, the macro, micro, and nanostructures of biomaterials play a vital role in determining their performance. This impact is evident not only in their physical and chemical properties, such as mechanical strength and porosity, but also in the behavior of cells, showcasing beneficial biological effects[5,6]. For example, the multi-layer structure of the scaffold has been prepared to achieve the gradient mechanical strength, which is necessary for hierarchical tissue repair[7]. The hierarchical or tubular structure is conducive to cell encapsulation and distribution, which is crucial for repairing tissue defects with characteristic structures such as tracheal defect repair and osteochondral regeneration[8]. In addition to the positive effect of the macrostructure of scaffolds on tissue regeneration, the corresponding micro/nanostructures of biomaterials are also critical[9]. For instance, inorganic biomaterials, such as zeolites, with different pore structures exhibit variable physical and chemical properties, affecting the drug loading efficiency[10]. Correspondingly, the differently structured zeolites with controllable loading rates not only serve as excellent drug delivery agents but also facilitate the adsorption of beneficial proteins to promote tissue regeneration. Moreover, Cells usually come into contact directly with biomaterials. Consequently, the micro/nanostructures of biomaterials can provide physical and chemical signals, further guiding cell behaviors in terms of cell adhesion, proliferation, morphology, and migration[11]. Overall, the structures are crucial in tissue regeneration, which indicates that the design of biomaterials with hierarchical structures is of great significance.
Hierarchically structured scaffolds are characterized by a combination of structural units that have distinct differences in their characteristics or components, following a certain regular or hierarchical pattern[12]. Examples of such scaffolds include multi-layered scaffolds, scaffolds with integrated units, and scaffolds with varying length scales, among others. The design of hierarchical structures is highly diverse and can be tailored to meet specific needs. It is worth noting that many tissues in the human body, such as osteochondral tissue, skin, and trachea, possess a hierarchical structure. For example, osteochondral tissue has a complex hierarchical structure, and its biology, physiology, and mechanical properties vary significantly throughout the thickness from the subchondral bone area below to the transparent cartilage area on the joint surface[13]. This suggests that compared to homogenous scaffolds, scaffolds with a hierarchical structure are expected to mimic the structure of native tissues better. Moreover, a hierarchically structured scaffold can provide an appropriate environment for cells to achieve optimal cell growth and function by offering ideal and variable mechanical support and physiochemical signal regulation[14]. Furthermore, a desirable hierarchically structured scaffold should closely resemble the target tissue in terms of its chemical and structural composition, ultimately leading to optimal tissue regeneration.
The hierarchically structured biomaterials with an integrated combination of macro, micro, and nanostructures have been developed to improve the efficiency of tissue regeneration. The physicochemical properties and bioactivities are enhanced due to the reasonable and fascinating design of hierarchically structured biomaterials. Generally, the macroscopic structure of materials influences the mechanical properties of scaffolds[15]. Hence, the macroscopic structure can be flexibly adjusted according to the mechanical strength demands of different tissues. The introduction of micro/nanostructures not only influences the mechanical properties of biomaterials but also improves their degradation behavior, which is convenient for the loading and releasing of drugs and growth factors[16]. Furthermore, the porous structure of scaffolds can influence cell infiltration and the transport of nutrients and metabolic products[17]. In contrast, the surface morphology that cells contact can further guide cell adhesion, proliferation, differentiation, and spreading[18], indicating the comprehensive effects on cell behaviors between the microporous structure and surface morphology of biomaterials. Likewise, the micro/nanostructures can improve extracellular matrix (ECM) deposition, cell alignment, and morphology[19]. The improved cell behaviors can regulate cell activity and microenvironments, leading to cellular functional responses[20]. Besides, attributed to the structure properties of biomaterials, the cell-cell interactions can be enhanced for tissue regeneration[21]. Overall, the integrated functions of macro and microstructures of hierarchically structured biomaterials play an important role in tissue regeneration.
In this review, we aim to summarize the recent progress on hierarchically structured biomaterials for tissue regeneration, focusing on the preparation strategies of hierarchical structure and their roles in regulating physicochemical properties and bioactivities [Figure 1]. The favorable effects of hierarchically structured biomaterials on tissue regeneration and the comprehensive functions of macro and micro/nanostructures are emphasized. Moreover, this review presents the recent applications of hierarchically structured biomaterials on tissue regeneration. Finally, the challenges that developments of biomaterials with hierarchical structures will encounter are discussed, aiming to provide cues for subsequent research.
DESIGN STRATEGIES AND PREPARATION METHODS OF BIOMATERIALS WITH HIERARCHICAL STRUCTURES
The performance of biomaterials in tissue regeneration can be regulated by changing the structures, which has stimulated the emergence of design strategies for different hierarchical structures. Biomaterials with hierarchical structures have been developed through three-dimensional (3D) printing, electrospinning, freeze-drying, etc.[22-25]. The hierarchical porous structures of biomaterials can be well designed by changing the corresponding parameters of these methods. In this section, we focus on summarizing these common preparation methods for developing hierarchically structured biomaterials, highlighting the diversity and importance of structure design for biomaterials.
3D printing
Currently, 3D printing has been applied as an essential method for manufacturing biomaterials. This technology can be used to prepare porous scaffolds by layer-by-layer (LBL) stacking[26], which is widely known and employed for its flexibility and convenience. As a practical method, 3D printing supports the design of hierarchically structured biomaterials. It can easily achieve the design from macroscale to microscale and accurately control the scaffold structures by changing related preparation parameters such as the nozzle and designed model[27]. For example, scaffolds with different pore distributions and sizes prepared by 3D printing possess excellent mechanical strength and convenience for cell growth and transportation, facilitating tissue regeneration[28]. The representative studies of using 3D printing techniques to fabricate scaffolds with hierarchical structures have been summarized in Table 1.
The hierarchically structured scaffolds fabricated by 3D printing for tissue regeneration
The types of 3D printing | Scaffold types | Application | Ref. |
Extruded 3D printing | Multi-layered scaffolds | Osteochondral tissue | [7] |
Scaffolds with integrated units | Bone | [32] | |
Scaffolds with varying length scales | Bone | [31] | |
DLP 3D printing | Multi-layered scaffolds | Cornea | [171] |
Scaffolds with integrated units | Bone | [35] | |
Scaffolds with varying length scales | Bone | [34,36] | |
Bioprinting | Multi-layered scaffolds | Wound healing | [38] |
Scaffolds with integrated units | Cartilage | [40] | |
Scaffolds with varying length scales | Muscle | [39] |
Extruded 3D printing
Extrusion 3D printing is one of the most commonly employed printing methods. Under the pre-set Computer-Aided Design (CAD) program, the printing nozzle extrudes ink based on biomaterials and stacks them LBL, forming continuous fibers to build the structure[29]. Assisted by this printing method, biomaterials can form 3D scaffolds with specific microstructures, demonstrating improved mechanical and biological properties for tissue regeneration[30]. For instance, our group constructed a flexible bioceramic scaffold with a spicule-like concentric layered bionic microstructure by extrusion 3D printing[31]. The shear stress field during the extrusion process could be adjusted by printing parameters, thereby regulating the concentric layered microstructure. Therefore, the designed bioceramic scaffold exhibited an ordered porous structure at the macroscale. At the same time, at the microscale, its pillars revealed concentric layered structures, accurately mimicking the layered structures of sponge skeletons and spicule. The scaffold possesses excellent flexibility, higher bending strength, and toughness, which could effectively solve the problem of high brittleness in traditional bioceramics and contribute to efficient bone tissue regeneration [Figure 2]. In another study, Zhang et al. designed a gradient hydrogel scaffold with a structure similar to osteochondral tissue by 3D printing[7]. The proportion of nano-sized hydroxyapatite in the three-layer scaffold increases sequentially, corresponding to the cartilage layer, calcified cartilage, and subchondral bone. Interestingly, the size of micropores also varied in gradient with the content of nano-sized hydroxyapatite. Therefore, the scaffolds with a gradient pore size range tended to promote chondrocyte activity and ECM formation, which was very beneficial for the construction of cartilage tissue. Compared to scaffolds with homogenous pore structures, this pore gradient could provide satisfactory mechanical support and optimize the repair of osteochondral defects in vivo. In addition, hydrogel/polycaprolactone (PCL)/SrCuSi4O10 (wesselsite, CS) core/shell scaffolds had been successfully prepared by coating PCL + CS on the surface of 3D-printed hydrogel scaffolds[32]. The core/shell structures could provide enhanced mechanical support for defects in the early stage. Afterward, the degradation of hydrogel led to the transformation of the core/shell scaffold into a hollow channel scaffold. The hollow channel structures could endow bone formation and angiogenesis by providing more space for cell growth. Therefore, the above studies demonstrated the important role of 3D printing in the preparation of hierarchically structured materials.
Figure 2. The fabrication of bioceramic scaffolds with spicule-like concentric layered bionic microstructures by extrusion 3D printing. (A) The schematic process of preparing spicules-inspired flexible bioceramic-based scaffolds by 3D printing. (B) Radial and axial morphology of the scaffolds printed by different printing nozzle lengths. (C) The scaffolds showing desirable manipulation and applicability. (D) Flexural strength of three groups. (E) VG staining of different groups. Reproduced with permission[31]. Copyright 2022, IOP Publishing Ltd.
Digital laser processing-based 3D printing
Digital laser processing-based (DLP) 3D printing is a forming technique that mainly solidifies photosensitive polymer liquids LBL through selective light irradiation[33]. The high molding accuracy of this technology is conducive to fabricating 3D scaffolds with complex and precise structures. Zhang et al. developed a type of large porous hydroxyapatite bioceramic scaffolds by DLP 3D printing[34]. The bioceramic possessed a micro/nanoporous surface structure, which could be finely adjusted. The in vitro experimental results showed that the designed bioceramics exhibited desirable bioactivity and the ability to promote bone regeneration. It was worth noting that the high-precision design and large-scale preparation of pore structures and surface morphologies of the scaffold could be achieved by DLP 3D printing. In another study, DLP 3D printing was applied to successfully and accurately manufacture tricalcium phosphate/bioglass composite (TCP/BG) scaffolds with helical structures[35]. Compared with commercial artificial bone graft materials, TCP/BG scaffolds with spiral structures could effectively induce bone ingrowth and integration. Furthermore, Zhang et al. manufactured macro/micro layered porous scaffolds by DLP printing to promote the ingrowth of bone tissue[36]. The micro/nano surface structures of hierarchical porous scaffolds were regulated into three different morphologies. The results of rat skull defect repair indicated that layered scaffolds with micro/nano surface structures were able to promote the differentiation of rabbit bone marrow mesenchymal stem cells (rBMSCs), exhibiting fascinating bone regeneration capabilities.
Bioprinting
At the forefront, 3D bioprinting involves printing living cells and bioinks together according to a predetermined structure to form a 3D living construction[37], which can be used in tissue engineering.
In articular cartilage, type II collagen arranged in an arched shape could form the main fiber network. Hence, it was reported that arched structures could be manufactured using two types of bioinks: GelMA and silk fibroin gelatin[40]. The arched structure is beneficial for promoting the chondrogenic gene expression of loaded cells by activating the transforming growth factor- β (TGF-β) signaling pathway, which plays an important role in forming fibrous collagen networks and cartilage formation.
Electrospinning
Among the different types of scaffold manufacturing technologies, electrospinning is the simplest and most versatile. It can be applied to construct scaffolds with biomimetic components and structures[41]. Nanofibers with different arrangements or surface structures can be obtained by adjusting electrospinning parameters and collection devices[42,43]. Subsequently, the obtained nanofibers can be further modified to endow them with further structures, indicating the feasibility and superiority of electrospinning in preparing hierarchically structured biomaterials[44]. For instance, Gan et al. successfully manufactured a type of 3D PCL/polylactide (PLA)/carbon nanotubes (CNTs) disk scaffolds with simple electrospinning technology[45]. At first, PCL/PLA electrospun fibers were formed and arranged in parallel or circular directions, corresponding to the collagen arrangement in the middle and peripheral areas of natural intervertebral discs. Then, the dual concave structure and regionally anisotropic microstructure recapitulated the structure of the native disc [Figure 3A and B]. The results of in vivo experiments demonstrated that the scaffold can guide intervertebral disc regeneration and protect subchondral bone. Han et al. applied the electrospinning method to construct nanofiber scaffolds with aligned core-shell structures to simulate the microstructure of natural annulus fibrosus tissues[46]. Nanofiber scaffolds could protect drugs in the core due to their unique core-shell structure. As the shell polymer dissolved, the drugs in the core were slowly released, meeting the requirements of “sustained release”. Meanwhile, the scaffold with an aligned microstructure, where the arrangement of annulus fibrosus cells was aligned with the direction of the nanofibers, was more similar to the normal physiological state of the annulus fibrosus cells. This hierarchical structure promotes annulus fibrosus tissue repair. Researchers have further improved traditional electrospinning techniques to develop more desirable structures[47]. Wang et al. combined melt electrospinning writing with solution electrospinning to prepare hierarchical scaffolds for bone tissue engineering with a layered structure of microfiber grid structure and random nanofiber morphology[48]. The designed layered scaffold provides mechanical support and a favorable microenvironment for cells to promote osteogenesis.
Figure 3. The preparation methods of biomaterials with hierarchical structures. (A) Schematic diagram of the preparation of a porous scaffold by electrospinning[45]. Copyright 2022, Acta Materialia Inc. Published by Elsevier Ltd. (B) The micro morphologies of 3D biomimetic electrospinning scaffolds[45]. Copyright 2022, Acta Materialia Inc. Published by Elsevier Ltd. (C) Preparation and application diagram of hierarchical chitosan scaffolds by freeze drying[58]. Copyright 2021, Wiley-VCH GmbH. (D) Schematic diagram of the manufacturing process of micro gel with core-shell structures and 3D printing scaffolds with micro gel loading cells[62]. Copyright 2021, Elsevier Ltd.
In some situations, the biomaterial structures fabricated by single electrospinning technology may not be enough for the specific requirements of tissue regeneration. Therefore, the design strategy of combining electrospinning with other techniques has been developed. Liu et al. prepared an anisotropic multi-scale cardiac scaffold by combining electrospinning and 3D printing[49]. The scaffold framework could mimic the interwoven myocardial anatomical structure, while the aligned electrospinning nanofiber network is able to guide cell alignment in a targeted manner. Moreover, a study was reported in which a nerve catheter was designed with poly(lactide-co-ε-caprolactone) (PLCL) nanofibers by electrospinning and gelatin hydrogel by 3D printing[50]. The formed PLCL nanofilms possess a transparent pore structure and can be applied as flexible external nerves for nerve conduits.
Meanwhile, the internally arranged paths were manufactured to produce patterned gelatin hydrogel with the surface microgroove, which can be used as the guiding path for effective axonal regeneration. Correspondingly, the experiment of sciatic nerve defects in rats demonstrated that PLCL/gelatin catheter could improve axonal regeneration. In addition, Zheng et al. developed an anisotropic micro/nanocomposite scaffold with electrospinning and micro-forming technology[51]. The scaffold presented a composite topology structure, with nanofibers randomly oriented and micro ridges/grooves parallel oriented. Their results indicated that the scaffold with anisotropic micro/nanocomposite structures not only enhanced mechanical properties but also guided the oriented growth of Schwann cells and promoted nerve regeneration.
Freeze-drying
Freeze-drying, known as ice templating, is a commonly employed preparation technique[52]. Freeze-drying first solidifies solutions, suspensions, sols, or gel under controlled conditions. After that, the solidified solvent template is removed by sublimation while the structural framework is retained, finally producing a well-shaped whole[53]. Freeze-drying is suitable for assembling units with different structures as long as these units are stably dispersed[54]. Its main advantage is that the macro/microstructures of the scaffolds can be regulated by changing the processing conditions[55]. This method is effective for the preparation of hierarchically structured biomaterials. For instance, 3D printing scaffolds with controlled macro/microstructures had been prepared by freeze-drying[56]. It was worth noting that changing the temperature and pressure of the drying progress could regulate the size of pillars and channels of the scaffold, thereby improving its mechanical performance and influencing the differentiation of seeded chondrocytes. Li et al. manufactured porous chitosan conduits with micro-patterned inner walls and seamless side walls using freeze-drying and microfabrication processes[57]. The results showed that chitosan conduits with micropatterned inner wall structures could promote peripheral nerve regeneration effectively compared to conduits without micropatterned structures. In another study, a novel layered chitosan scaffold was prepared to accommodate different types of cells and promote gingival tissue regeneration by bidirectional freeze-drying[58]. This scaffold possessed a typical long-distance porous layered microstructure, which could simulate the layered structure of attached gingiva and provide superior mechanical properties, contributing to the regeneration of soft gingival tissue [Figure 3C].
The combination of multiple fabrication methods
For the precise and complex preparation of hierarchically structured biomaterials, a single preparation method still has limitations, which need the participation of multiple fabrication methods. Therefore, the employment of multiple preparation methods together to construct biomaterials with hierarchical structures has been widely studied. Among them, the combination of 3D printing and other methods is often used. Our group prepared scaffolds with controllable micro/nanostructures through 3D printing and hydrothermal methods[59]. The scaffolds were prepared by 3D printing, and then micro/nano calcium phosphate crystals with different morphologies were successfully grown on the surface of the scaffolds through the hydrothermal method. The macroscopic porous structure of the scaffold provided mechanical support and cell transport for tissue regeneration. In addition, the introduction of microstructures further improved mechanical properties and could promote the migration and differentiation of chondrocytes, regulating cell morphology. The hierarchical structures formed by these two methods provide comprehensive and satisfactory effects for osteochondral regeneration. Besides, Yin et al. developed a high-density polyethylene scaffold with satisfactory pore size and connectivity[60]. The microstructure of this scaffold could be improved by the LBL method to enhance its bioactivity and antibacterial performance. Among them, the macro and microstructures were adjusted through 3D printing parameters. A multilayer
THE FUNCTION OF BIOMATERIALS WITH HIERARCHICAL STRUCTURES FOR TISSUE REGENERATION
Both the macro and microstructures are able to regulate the physicochemical properties and bioactivities of biomaterials, finally facilitating tissue regeneration[63]. In this section, we summarize the function of biomaterials with hierarchical structures on tissue regeneration, focusing on their role in tissue regeneration by influencing the physicochemical properties and bioactivities of biomaterials.
The improvement of physicochemical properties
The physicochemical properties of biomaterials are essential for tissue regeneration[64]. For instance, appropriate mechanical properties can provide sufficient support before synthesizing newly formed bones, which is beneficial for bone growth. Different bone defect sites have additional requirements for mechanical strength[65]. The degradability of scaffolds also requires reasonable regulation to meet the needs of varying tissue regeneration[66]. Meanwhile, adjusting other physicochemical properties is also favorable for tissue regeneration[67]. Therefore, designing hierarchical scaffolds to regulate and improve these physicochemical performances is crucial for tissue regeneration.
Improved mechanical performances
Matching the mechanical properties of materials with tissue defects is an essential part of tissue repair. The design of different hierarchical structures can play an important role in regulating mechanical performances[68]. Therefore, increasing studies concentrate on regulating the mechanical properties of scaffolds by designing hierarchically structured scaffolds.
Among the structural clues provided by biomaterials, porosity is one of the main factors regulating the mechanical properties of the scaffold[69]. For example, our group developed a type of Haversian bone-mimicking scaffold with a layered Haversian bone structure through DLP-based 3D printing[70]. By changing the parameters of the Haversian bone simulation structure, the porosity of the scaffold could be well controlled, thereby affecting the compressive strength of the scaffold [Figure 4]. Besides, Jia et al. prepared layered porous Mg scaffolds with customized interconnectivity and pore size distribution[71]. Among them, porosity and pore size could change the mechanical strength of the scaffolds, and the scaffolds with better interconnectivity showed lower mechanical strength. This study indicates that the design of interconnectivity and pore size distribution can provide a flexible strategy to achieve mechanical matching with the host organism. Moreover, the macroscopic structure of the scaffold, combined with the influenced surface curvature and surface area, can also affect mechanical strength. Zhang et al. constructed a 3D-printed hydroxyapatite scaffold based on triply periodic minimum surface (TPMS) structures[72]. The results indicated that the hydroxyapatite scaffold based on the TPMS structure possessed a more extensive range of compressive strength due to the variable surface area and microporous structures. The changes in the microstructures of hierarchical biomaterials can also influence the mechanical properties of scaffolds. For instance, Meng et al. developed poly(L-lactic acid) (PLLA)/PCL nanofiber scaffolds with heterogeneous porous structures[73]. A part of PCL was extracted from the fibers and enriched on the fiber surface, which changed the surface morphology and characteristics of the scaffold. Fascinatedly, the experiment results indicate that these heterogeneous porous nanofiber scaffolds revealed excellent mechanical properties. Besides, in order to guide periodontal tissue regeneration effectively, a study was conducted on the preparation of bilayer membranes with different superimposed surfaces[74]. The differences in surface morphologies, including fiber arrangement and roughness, had improved the mechanical properties of membranes and were expected to serve as guiding periodontal regeneration scaffolds. In addition to the above research, our group also explored the compressive strength of the scaffolds by endowing them with different structural characteristics on the surface[59]. The results showed that the growth of different micro/nano calcium phosphate morphologies on the surface of the scaffolds could significantly improve the compressive strength by repairing micro-cracks.
Figure 4. The regulation of mechanical performances by the structures of scaffolds. (A) Optical microscope images exhibiting different diameters and numbers of Haversian canals. Scale bars, 1 mm. (B) Micro-CT images of Volkmann canals (blue arrows) connecting Haversian canals in the interior of scaffolds. Scar bars, 1 mm. (C) Micro-CT images of scaffolds with different numbers of Haversian canals. Scar bars, 1 mm. (D) The compressive strength of scaffolds with different numbers of Haversian canals. (E) Porosity of scaffolds with different numbers of Haversian canals. (F) Micro-CT images of scaffolds with different diameters of Haversian canals are indicated by blue arrows. Scar bars, 1 mm. (G) Compressive strength of scaffolds with different diameters of Haversian canals. (H) Porosity of scaffolds with different diameters of Haversian canals. (I) Micro-CT images of scaffolds with different numbers of Volkmann canals. Scar bars, 1 mm. (J) Compressive strength of scaffolds with different numbers of Volkmann canals. (K) Porosity of scaffolds with different numbers of Volkmann canals. Reproduced with permission[70]. Copyright 2020, American Association for the Advancement of Science. *P < 0.05, **P < 0.01, ***P < 0.001.
The hierarchical structures of biomaterials display variable mechanical properties in different areas, which can match the varying mechanical characteristics of the tissue defects. For example, Fan et al. prepared frozen gel with an anisotropic structure to accelerate tissue regeneration[75]. The periphery of the cryogel scaffold was a relatively dense layered structure to imitate the cortical bone, while the inner layer possessed a uniform porous structure. The inconsistent porosity at different levels led to varying mechanical properties, which could provide further biomechanical clues to achieve rapid bone healing. Besides, Li et al. designed a biomimetic scaffold with a heterogeneous porous structure for the regeneration of the rabbit humeral head[76]. The cartilage and skeletal areas of this scaffold are composed of different supporting materials. The design of this heterogeneous structure would enable the scaffold to exhibit coordinated dynamic mechanical stimulation characteristics, promoting endochondral ossification and leading to effective subchondral bone regeneration.
It is necessary to simulate the microstructure and mechanical properties of natural tissues to achieve rapid tissue regeneration. Therefore, there was a study constructing a layered spiral scaffold with high tensile strength, which was similar to the structure of natural tissues and matched with their non-affine deformation mechanical properties[77]. Under the synergistic effect of elastic polymer and spring coil structure, the spiral scaffold revealed desirable tensile properties. Meanwhile, the local cell stretching bore much less pressure than the overall scaffold, creating a stable mechanical microenvironment for new tissue growth. Ligament regeneration is a complex process. Designing a hierarchically structured scaffold is expected to achieve dynamic mechanical performance and meet the needs of ligament regeneration[78].
Degradability
In the application of tissue regeneration, biodegradability is one of the important evaluation indicators for the application efficiency and value of biomaterials[80]..In order to satisfy the regeneration speed of different tissue repairs, the degradability of biomaterials needs to be reasonably regulated. Many studies have shown that the structures of biomaterials, especially the presence of hierarchical structures, can significantly affect their degradability[81,82]. For example, the hierarchical porous structure of biomaterials can not only achieve regulation of degradability but also cause the overall scaffold to exhibit inconsistent degradation rates, which is beneficial for tissue regeneration with an uneven structure[83]. In addition, hierarchical structures can flexibly regulate the degradability of different levels, resulting in the variable release rate and sequence of bioactive ions or loaded drugs and meeting the diverse need for tissue regeneration[84]. Therefore, it is worth paying attention to the improvement and regulation of hierarchically structured biomaterials on biodegradability.
The pore structure characteristics, including porosity, pore size, and pore connectivity, can affect the degradability of biomaterials. Kim et al. designed a magnesium phosphate ceramic scaffold with a multi-level pore structure[85]. Regulating the diameter of the micropores inside the scaffold formed structures with different porosity, which resulted in a regular change in the degradation rate. Magnesium phosphate scaffolds with higher porosity promote faster biodegradation and then enhance the formation and remodeling activity of new bones. Shi et al. fabricated bioactive glass scaffolds with two distinct architectures while maintaining a constant interconnection size of the scaffold pore structure but with different porosity. The degradation experiment results indicate that the degradation rate of scaffolds with higher total porosity was higher, which allowed for rapid bone ingrowth and cancellous bone formation[86]. Furthermore, some studies have shown that the geometric shape of pores can also affect the biodegradability of scaffolds. Li et al. designed three types of scaffolds with different pore shapes, namely gyroscopes, cylinders, and cubic pores[87]. The in vitro degradation experiments showed that the cylindrical porous scaffold had a slower mass loss in the first eight weeks. Besides, the release rate of calcium and silicon ions of cubic porous scaffolds in the early stage was slightly slower than other scaffolds.
Tissue regeneration proposes higher requirements for the degradability of scaffolds. During tissue regeneration, the uniformity of scaffold degradation will lead to part tissues being difficult to ingrow, thereby negatively affecting the efficiency of repair[88]. In addition, the degradability of biomaterials can affect the release behaviors of loaded growth factors or drugs. Dynamic and controllable release behavior is more conducive to tissue repair or disease treatment[89]. Fortunately, the design of the hierarchical structure provides a solution for these requirements, which can contribute to dynamic or controllable degradation of scaffolds. Dou et al. prepared hierarchical scaffolds with graded pore structures, demonstrating gradient degradation performance[90]. The internal microporous structure was able to rapidly degrade and provide an open space for the ingrowth of cells and tissues. At the same time, the overall structural integrity of the scaffold remained for at least 32 weeks, endowing reliable mechanical support for tissue growth. The degradation differences caused by hierarchical structures can also lead to variations in the loaded factors or release sequence of drugs and behaviors of the scaffold. For example, the layered porous scaffold prepared by Wang et al. achieved sequential release of angiogenic peptides (AP) and osteogenic peptides (OP) loaded in different structures due to varying degradation behaviors of the whole scaffold. AP exhibited rapid release behavior, and OP release was slow but continuous[91]. Zhou et al. assembled the prepared short nanofiber dispersion into the pores of the 3D printing scaffold to obtain a scaffold with a flower bed-like structure[92].
Attributed to the different degradation rates of nanofibers and 3D-printed microfilaments at various structures, dimethyloxalylglycine (DMOG) and Sr ions can be sequentially released from the biomimetic scaffold to achieve timely vascularization and stable bone formation. Likewise, 3D-printed gradient scaffolds with different pore sizes were prepared and loaded with E7 peptide and B2A peptide, respectively[93]. The site E7 peptide loaded exhibited rapid degradation behavior, which can lead to quick release of E7 peptide. Meanwhile, the slowly degraded silk fibroin porous matrix sustained the release of B2A peptides. Benefiting from programmed biomolecular delivery caused by differences in spatial structural degradability, this scaffold displayed enhanced cartilage and subchondral bone regeneration. Furthermore, there was a study that showed by designing the geometric structure of hierarchical microdevices, the degradation behaviors could be regulated, thereby accurately controlling the drug release behaviors[94].
The influence on other physicochemical properties
The structures of biomaterials have a significant impact on mechanical properties and degradability. To benefit tissue regeneration, they can improve some other physicochemical properties of the scaffold, such as hydrophilicity, permeability, roughness, and conductivity. Our group employed bamboo with a layered porous structure as a template and combined biomimetic mineralization method to prepare mineralized calcium phosphate/bamboo composite scaffolds[95]. Owing to the hierarchical porous structure of bamboo templates and bioactive mineralized components, mineralized biomaterials exhibited enhanced hydrophilicity and liquid transport performance, which could promote cell transport and nutrient diffusion during tissue regeneration. Besides, a bilayer scaffold with a hierarchical structure similar to human skin was developed using silk fibroin and sodium alginate[96]. The upper membrane showed a poreless structure, while the lower scaffold possessed a porous structure. The results found that the scaffold revealed appropriate water permeability, moderate hydrophilic surface, and suitable water vapor permeability, which could provide a moist environment for wound healing and protect them from dehydration. The surface roughness of the material can affect the adhesion of the scaffold to cells. A study constructed carbon-based scaffolds with hierarchical nano- and micro-scale structures, which could control physical and chemical properties[97]. Microscale features (interconnecting pores and arranged fibers) and surface functionalized nanostructures could precisely regulate the nano roughness and wettability of scaffolds, thus profiting cell adhesion and differentiation. The regeneration strategy of tissues, such as nerves, muscles, and the heart, requires biomaterials with certain conductivity[98]. A study was conducted on coating various layers of Mxene on the surface of PCL fiber scaffolds to form composite scaffolds with diverse surface morphologies[99]. The presence of defective structures and Mxene layers endowed the possibility of this scaffold as a conductive biomaterial for the application of tissue regeneration. In addition, changes in surface structure caused an increase in the surface activity of the scaffold, providing a favorable environment for cell adhesion and proliferation.
The improvement of bioactivities
Correspondingly, the bioactivities of biomaterials are also important for tissue regeneration, which can influence the behavior of cells, thereby regulating related functions such as angiogenesis, neurogenesis, and anti-inflammatory[100]. It is worth noting that the macro and microstructures of biomaterials can guide cell adhesion, morphology, migration, proliferation, and differentiation by providing physical and chemical signals[101]. In addition, the micro morphologies offered by the structure have positive effects on particular biological functions, including angiogenesis, neurogenesis, immune regulation, and improving cell metabolism. For example, the morphology from submicron to nanoscale can directly affect cells, and submicron morphology can have an impact on intercellular interactions, intercellular signal transduction, and other cellular activities[102]. Moreover, some reports have shown that the nanopore structure can alter the conformation of certain cell attachment proteins or affect cell function by altering surface energy[103]. Therefore, in this section, we focus on summarizing the bioactivities of hierarchical biomaterials with different macro and microstructures in guiding tissue regeneration from three aspects: regulating cell behaviors, improving cell functions, and fostering intercellular interactions.
The regulation of cell behaviors
Cells adhesion. Cell adhesion is an important cellular process in the formation of new tissues. The macro and microstructures of biomaterials can regulate cell adhesion by providing physical and chemical signals such as surface area, hydrophilicity, surface roughness, and mechanical stimulation[100]. The surface area regulated by structure regulation can affect cell adhesion sites. Zhang et al. investigated the effects of nanorod arrays on the front surface and honeycomb-like microstructures on the adhesion behavior of stem cells[104]. The results showed that the adhesion area of stem cells in the honeycomb microstructure was much smaller because the diameter of the honeycomb structure was relatively large, leading to cells falling into pores and limiting their adhesion range[104]. In addition, a multi-level micro/nanostructure scaffold was prepared to investigate the effect of different mesoporous pore sizes of the scaffold on cell adhesion. The results showed that the larger mesoporous pore size was conducive to protein adsorption due to its larger specific surface area, thereby promoting cell adhesion[105].
The regulation of hierarchical structures on the hydrophilicity of scaffolds has been analyzed. Hydrophilicity also has a certain impact on cell adhesion. Research has found that moderately hydrophilic surfaces can enhance cell adhesion. In the study of osteoblasts, cell adhesion increased after the improvement of hydrophilicity[106]. Hou et al. prepared gradient surfaces with morphologies ranging from nanoscale to micrometer scale roughness[107]. The optimized layered structure, combined micron and nanoscale roughness, showed an increased surface area that facilitated protein adsorption to enhance the affinity between cells and substrates. Further research demonstrated that different surface roughness could regulate cell adhesion through related mechanical stimulation pathways, including signal pathway activation and nuclear tension generation.
Cells arrangement. The orderly or controllable arrangement of cells in various tissues plays a vital role in maintaining specific functions[108]. Therefore, designing scaffolds with specific structures to guide the arrangement of cells is meaningful. Generally, to guide the arrangement of cells, the scaffold needs to mimic the structure of native tissue. Current research indicates that specific structures can promote cell orientation[109]. However, a single structural characteristic limits the accuracy and efficiency of cell orientation due to the complexity of native tissues. Hierarchical structure design is expected to combine various structures required for cell arrangement and can also regulate the physical and chemical gradients involved in forming ECM to control cell behaviors, thereby highlighting cell functions and promoting tissue regeneration more effectively[14]. In a study, silicon molds with four types of surface morphology, including hemispherical, pyramid, semi-cylindrical, and triangular microstructures, were transferred to the surfaces of PCL films and nanofiber scaffolds, respectively. The results of in vitro experiments indicated that triangular microstructure nanofibers could guide the longitudinal elongation and arrangement of cultured C2C12 myoblasts, forming slender cell morphology[110]. In addition, Yu et al. constructed a scaffold with a layered double-layer structure. The prepared biphasic scaffold revealed a unique micro/nanostructure and stable two-phase interface[111]. The lower layer exhibited a periodontal ligament-like periodic parallel arrangement structure, promoting the migration and orientation of endogenous stem cells, thereby supporting the parallel arrangement of newly formed collagen fibers. Moreover, a study controlled the macroscopic cell arrangement by adjusting the microstructure of the scaffold[112]. It was found that square grids showed randomly oriented cells. In contrast, the arrangement of cells in diamond grids was anisotropic, where cells were arranged along the main axis of the diamond shape along a single axis.
Cells migration. The hierarchical structures of biomaterials are also effective in promoting cell migration. Our group designed conch-like scaffolds with a hierarchal structure. The internal scaffold was comprised of a spiral cross-section, a cavity, and an intermediate support spar, while the external showed the outer walls containing penetrating macropores[113]. Interestingly, the spiral structure of the scaffold could effectively induce cells to migrate from the bottom to the top. Hence, attributed to the directional migration of cells in the conch-like scaffold, the scaffold could effectively guide the growth of bone tissue from the bottom to the top [Figure 5]. Besides, Yang et al. prepared hydroxyapatite scaffolds with three different structure types (tortuous channels, parallel channels, and gradient channels) by freeze-drying[114]. The results showed that graded channels could significantly enhance cell migration compared to tortuous or parallel channels, as graded channels promoted fluid circulation inside the scaffolds by the “capillary effect”, accelerating metabolic waste clearance and nutrient transport[115]. The regulation of pore structures on cell migration is also critical. Composite scaffolds with randomly arranged, radially arranged, or axially arranged pores were developed to investigate the effects of different pore structures on the migration of cells[116]. The results revealed that radially and axially arranged scaffolds could promote the migration of endogenous repair cells in cross-sectional and vertical directions, respectively.
Figure 5. The hierarchically structured biomaterials promote cell migration. (A) The adhesion of rBMSCs in 3D-printed conch-like scaffolds with different spacing. (B) Migration images of rBMSCs in 3D-printed conch-like scaffolds with different spacing at various time points. Statistical analysis of cell migration in the Z direction (C) and XY direction (D). Reproduced with permission[113]. Copyright 2022, Publishing services by Elsevier BV on behalf of KeAi Communications Co. Ltd. *P < 0.05, ***P < 0.001.
Cells proliferation and differentiation. The proliferation and differentiation behaviors of cells are crucial for tissue regeneration, which can also be adjusted by the structures of the biomaterial. Thus, scaffolds with different surface microstructures were designed to investigate the regulatory effect of morphology on cell behaviors[117]. The results showed that when cells were cultured on nanogratings, the percentage of proliferating cells was significantly lower than that on flat surfaces and nanocolumns. Besides, nanogratings were beneficial for the adipogenic differentiation of human mesenchymal stem cells (hMSCs). Nanomorphology could regulate cell behavior by mechanically coupling the cell membrane and nuclear envelope through the cytoskeleton to deform the nucleus at the nanoscale. Considering the positive impact of micro/nano surface structure on cell bioactivity, scaffolds with different surface micro/nanostructures have been designed and investigated to improve bioactivities in tissue regeneration. Yang et al. developed a 3D porous scaffold with controllable nano morphologies on the pore wall surface by a surface modification process to enhance the cell bioactivity of BMSCs and promote bone repair[118]. The designed surface nanostructures included nanoneedles and nanosheets, which were only formed on the surface of the pillars. Subsequently, it was found that nanoneedle surface structures revealed more excellent bioactivity in promoting BMSC attachment and osteogenic differentiation. Moreover, our group proposed a microbial catalytic strategy to generate calcium carbonate with micro/nanostructures on the surface of bioceramic scaffolds[119]. Microbial-catalyzed micro/nanostructures were able to promote cell adhesion and osteogenic differentiation, demonstrating desirable bioactivities. It could be attributed to the influence of the physical and chemical properties of different micro/nanostructure surfaces on the expression of the integrin alpha-2, which could participate in cell-surface interactions and play an important role in subsequent osteogenic differentiation[120].
The impact of structures on a single type of cell behavior has been explored. However, the function of biomaterials on cell behaviors is comprehensive in tissue regeneration. The integrated functionality of hierarchical structure in improving cell behaviors should be emphasized because they can provide more structural characteristics, which are conducive to the comprehensive regulation of a series of cell behaviors[121]. For example, a micro/nano-hierarchical scaffold was constructed by incorporating arranged micro/nanofibers into layered scaffolds[122]. The design of this hierarchical structure significantly improved the proliferation and arrangement of myoblasts and even promoted the formation of myotubes. Likewise, Meng et al. combined 3D printing, electrospinning, and unidirectional freeze-casting to develop composite scaffolds with mechanically robust frameworks and aligned nanofiber structures[123]. The framework of the scaffold with a porous structure could guide cell infiltration and migration. Besides, the arranged nanofiber structure was conducive to cell adhesion, infiltration, and differentiation, which could be ascribed to the effect of increasing the contact area between cells and materials and the properties of fibers on cell morphology. Moreover, hierarchically ordered recombinant human collagen hydrogel was prepared with microstructures of inverse opal nano-scale pores and aligned microgrooves for corneal stroma regeneration[124]. The ordered microstructure could stimulate the orderly growth and differentiation into keratinocytes of limbal stromal stem cells in vitro, thereby supporting the regeneration of corneal stroma. Overall, the hierarchical structures of biomaterials can influence the interactions between cells and materials by providing unique physical and chemical clues, thereby achieving regulation of cell behaviors.
Promotion of specific cell functions
The hierarchical structure of biomaterials can regulate specific cellular behavior by providing certain physical and chemical clues, thereby improving cellular function[125]. Scaffolds with different fiber diameters and porosity were prepared to investigate the functions of microstructures on angiogenesis[126]. It was found that scaffolds with relatively low fiber diameter, higher porosity, and specific surface area could promote the formation of dense microvascular systems, demonstrating the feasibility and effectiveness of structure changes in regulating angiogenesis. Ha et al. manufactured bone microenvironment-mimetic scaffolds with hierarchical micro/nanofibers and microchannel structures[127]. The microchannel structure inside the scaffold was similar to vascular-like structures, with perfusion properties that facilitated nutrient transport and promoted gene/protein expression related to angiogenesis. Besides, the improved degradation characteristics could benefit drug release and further encourage angiogenesis.
Furthermore, the structure signals of biomaterials are also beneficial for neurogenesis in tissue regeneration. Lu et al. prepared neural conduits with a hierarchical anisotropic architecture[128]. The conduits could support the migration of Schwann cells and induce neural differentiation, confirming that the arrangement structure was beneficial for regulating the biological response of nerve cells to promote neurogenesis [Figure 6]. In addition, another study developed an aligned composite topography with micro-sized ridge/groove structures and nano-sized fibers together to promote neurogenesis[129]. The results showed that the scaffold could effectively induce the directed growth of Schwann cells and upregulate genes and proteins related to myelin formation.
Figure 6. The regulation of neurogenesis by hieratical structures. (A) Schematic diagram of the fabrication of neural conduits with a hieratical structure. (B) Optical photograph of hollow conduits. (C) The photo of conduits filled with aligned hydrogel. (D) SEM images of conduits filled with silk nanofiber fillers. Laser scanning confocal microscopic images (E) and proliferation (F) of Schwann cells cultured on different concentrations of layered conduits for one, three, and seven days. (G) The distribution of orientation angle of Schwann cell nucleus after seven days. (H) ELISA for brain-derived neurotrophic factor (BDNF) secreted by cells on hieratical conduits. (I) Longitudinal and transverse H&E staining of regenerated nerves in vivo. (J) S-100 and NF-200 staining of regenerated nerves at
The macro and micropore structures of biomaterials can regulate the immune microenvironment by improving the behavior of immune cells, which is beneficial for tissue repair[9]. Our group fabricated gear-inspired 3D bioceramic scaffolds with an ordered microstructure[130]. The research found that the scaffold could regulate the polarization of macrophages, exhibiting anti-inflammatory functions [Figure 7]. The possible mechanism by which the scaffold possessed good immune regulation function was due to the inhibition of macrophage contact by the microchannel structure, resulting in the loss of E-cadherin. The loss of E-cadherin in macrophages would lead to the translocation of β-catenin from membrane to nucleus, which would finally adjust the polarization of macrophages[131]. In addition, He et al. developed a novel hierarchical-structured mineralized nanofiber scaffold that combined mineralized arranged nanofibers (anisotropic) and mineralized random nanofibers (isotropic)[132]. It was found that the morphology of mineralized arranged nanofibers within the scaffold showed bone immune microenvironment regulation function, which could effectively stimulate the polarization of macrophages and promote the secretion of healing cytokines IL-4 and IL-10. In another study, three types of hierarchical porous scaffolds with different pore size combinations were designed to investigate the effects of varying levels of pore size on the inflammatory response of macrophages[133]. Different levels of pore structures could induce macrophage polarization into pro-inflammatory M1 macrophages in the early stage and efficiently switch to anti-inflammatory M2 macrophages in the later stage by influencing the morphology of macrophages. The hierarchical pore structure could quickly regulate the transition of macrophage inflammatory response, reduce pro-inflammatory response, and increase anti-inflammatory response, creating a more favorable bone repair microenvironment. The controlled regulation of macrophages enabled the tissue immune microenvironment to quickly recover to tissue homeostasis after tissue repair rather than interfering with the homeostasis of the host[134]. In addition, M2 macrophages could increase the survival rate and osteogenic differentiation of mesenchymal stem cells (MSCs) in bone regeneration and benefit angiogenesis[135]. These processes were essential for bone defect repair. Therefore, the hierarchical porous scaffolds were beneficial for tissue repair and regeneration by providing a satisfactory immune response mode.
Figure 7. The immune microenvironment regulated by pore structures and surface microstructures. (A) Optical images of gear-inspired 3D bioceramic scaffolds with ordered surface microstructures. (B) SEM images of scaffolds with different microstructure parameters. (C) SEM images of gear-inspired scaffolds with different microstructure shapes. (D) Laser scanning confocal microscopic images of RAW264.7 cells on the surface of different gear-inspired scaffolds. (E) The inflammation-related gene expression of RAW264.7 cells after cultured. (F) Masson trichrome staining of mouse skin and subcutaneously implanted scaffolds. (G) Micro-CT reconstruction model of newly formed bone (green) and implanted scaffold (red). Reproduced with permission[130]. Copyright 2022, Elsevier Ltd. *P < 0.05, **P < 0.01, ***P < 0.001.
Biomaterial structures can also regulate metabolic function due to relevant signaling pathways activated by influencing cell behavior. For instance, in order to meet the unique metabolic direction and the vast demand for nutrient delivery of flat bone regeneration, researchers have designed different configurations of flow channels in hydroxyapatite porous scaffolds[136]. Curved channels exhibited higher permeability, enhancing material transport and cell sedimentation by forming eddies. Flow channel scaffolds were able to provide a rich blood supply and cell/nutrient transportation, resulting in better tissue ingrowth ability. Particularly, the integration of curved and annular channels demonstrated comprehensive performance, promoting flat regeneration by improving metabolism in the repair microenvironment.
In addition, Gu et al. prepared scaffolds mimicking the surface structure of the liver extracellular skeleton for bone regeneration[137]. The research found that specific surface structures could activate related signaling, leading to Arg2 retrograde translocation from mitochondria to the cytoplasm. Translocation further promoted spermine and mitochondrial respiration, reprogramming energy metabolism and arginine metabolism in macrophages. Moreover, a micropatterned collagen scaffold with a corner layer structure simulating the microstructure features of Annulus fibrosus was fabricated[138]. It was found that the micropatterns on the surface of the collagen membrane regulated the morphology and orientation of BMSCs to a directional arrangement, and the aligned cells in the scaffold showed increased expression of genes and proteins related to matrix anabolism.
The regulation of cell-cell interactions
Cell-cell interactions, as fundamental biological processes of multicellular organisms, are crucial for growth, tissue formation, and intercellular communication[139]. Likewise, the structure characteristics of biomaterials also influence cell-cell interactions by providing physical and chemical signals. For example, Ren et al. prepared uniformly distributed PCL nanoneedle arrays, and the surface was modified with polydopamine[140]. After modification with polydopamine, compared to direct cultivation on nanoneedle arrays of cells, the cell state changed from aggregated distribution to laminar distribution. The laminar distribution of cultured cells led to weak intercellular interactions, weakening the pluripotency of stem cells, while the aggregation distribution of cells cultured on pure PCL nanoneedle array enhanced intercellular interactions, which was beneficial for maintaining the pluripotency of BMSCs.
Research results showed that large interconnections between different pores promoted cell communication, thereby facilitating the production of ECM[141]. In addition, a study employed nanoscale wavy surface morphology as the extracellular environment for cell culture. The results indicated that surface morphology could influence cell-cell interactions to make cells display different degrees of convergence, further regulating cell arrangement[142]. Moreover, isotropic-arranged nanowires were prepared to investigate the effects of structures on neurite growth and neuronal formation. The isotropic arrangement of nanowires activated mechanical conduction pathways as physical clues to guide the development of neurite and help form networks with neighboring neurons through intercellular communication of synaptic connections[143].
Meanwhile, the hierarchically structured biomaterials were very suitable for loading multiple types of cells to enhance the interaction between different kinds of cells, which is conducive to tissue regeneration. Inspired by plant chloroplasts, our group designed a dendritic scaffold as a 3D platform for multicellular delivery and multiple tissue interactions[144]. The multi-layer leaves of the scaffold fully supported the adhesion, proliferation, and osteogenic and neurogenic differentiation of BMSCs and Schwann cell co-culture systems. Besides, the gradient structure on the surface of scaffold leaves could promote the proliferation, osteogenesis, and neurogenic differentiation of the co-culture system by regulating layer thickness. Succulent plant-like bioceramic scaffolds had also been prepared to create beneficial cellular microenvironments for bone regeneration[145]. The succulent-like structures could prevent cells from leaking from the scaffold and enhance cell adhesion. They also possessed excellent cell loading and cell distribution regulation performance, promoting cell interactions and osteogenic differentiation of stem cells. Moreover, Zhang et al. applied Haversian bone-mimicking bioceramic scaffolds to establish a platform for regulating multicellular bone immune response and study the interaction between MSCs and macrophages[146]. The results showed that the immune response of macrophages was influenced by the proportion of MSCs, demonstrating the regulatory effect of hierarchically structured scaffolds on the spatial distribution and interaction between multicellular spaces[146]. Furthermore, our group designed a honeycomb-shaped scaffold with a parallel channel structure. By loading MSCs and macrophages into different corresponding microchannels, the scaffolds were constructed with different cell arrangement patterns (star shape, Tai Chi shape, and staggered shape to investigate the function of the designed bone immune microenvironment in tissue regeneration[147]. Correspondingly, this study also indicated that the structure of scaffolds can achieve “cross-talk” reactions between multiple cells, thereby providing different bone regeneration microenvironments.
THE RECENT APPLICATIONS OF HIERARCHICALLY STRUCTURED BIOMATERIALS FOR TISSUE REGENERATION
We have recapitulated the function and importance of hierarchically structured biomaterials in improving physicochemical properties and bioactivities above. In this section, the recent applications of hierarchically structured biomaterials for tissue regeneration are discussed. Figure 8 shows the schematic diagrams of the structures of some natural tissues. It can be found that most native tissue organizations have hierarchical structures. Therefore, hierarchically structured scaffolds possess significant advantages in promoting tissue regeneration.
Figure 8. The schematic diagrams of the hierarchical structures of different native tissues. (A) The structure schematic diagram of Haversian bone[70]. Copyright 2020, American Association for the Advancement of Science. (B) The histologic structure of an osteochondral unit[173]. Copyright 2020, Springer Nature. (C) The schematic of tendon-to-bone tissue[174]. Copyright 2020, Production and hosting by Elsevier BV on behalf of KeAi Communications Co., Ltd. (D) The diagram of skin[175]. Copyright 2019, WILEY-VCH Verlag GmbH & Co. KGaA, Weinheim. (E) The diagram of tracheal tissue[176]. Copyright 2020, John Wiley & Sons, Ltd. (F) The schematic diagram of periodontal tissue[177]. Copyright 2021, Publishing services by Elsevier BV on behalf of KeAi Communications Co. Ltd. (G) Scheme illustrating myocardial architecture[167]. Copyright 2019, WILEY-VCH Verlag GmbH & Co. KGaA, Weinheim. (H) Schematic diagram of the native muscle structure[168]. Copyright 2019, Elsevier Ltd. (I) Schematic diagram of human cornea[178]. Copyright 2020, Elsevier Ltd. JE: junctional epithelium; CT: connective tissue; PDL: periodontal ligament; CM: cementum; AB: alveolar bone.
Bone regeneration
Bone repair is a complex process that involves the reconstruction of biological functions and the restoration of mechanical properties. Recently, the strategy for repairing bone defects is to design bioactive scaffolds with specific functions to meet the needs of bone regeneration. Hierarchically structured biomaterials display great advantages in designing bone repair scaffolds due to their excellent regulation of physicochemical properties, including mechanical properties and enhanced bioactivities such as angiogenesis, nerve regeneration, and immune regulation.
Li et al. designed a Haversian-like hierarchical porous scaffold for bone regeneration[148]. The axial tunnel composed of peripheral large pores could form a Haversian tubular tunnel, and the central small pore was similar to the cancellous bone. Compared to uniform scaffolds, the gradient scaffold exhibited favorable mechanical properties and excellent bioactivities, such as promoting cell migration and angiogenesis, effectively supporting bone regeneration in vivo. Besides, a series of bioceramic scaffolds with different curved pore walls were manufactured to explore their potential applications in bone regeneration[149]. It was found that Gyroid and Diamond porous scaffolds could significantly induce osteogenic differentiation of BMSCs, supporting early bone tissue regeneration, owing to the pore characteristics of Gyroid and Diamond scaffolds being more conducive to bone conduction. Furthermore, Zhang et al. developed 3D printing scaffolds with a hierarchical porous structure[150]. This scaffold could mimic cortical and cancellous bone tissue. Meanwhile, the soft hydrogel infused through internal channels further endowed the scaffold with the features of ECM. Research results found that the layered structure contributed to integrating multiple functions into one platform. Finally, the induced comprehensive performance regulation endowed the scaffold with desirable capacities for immune regulation, angiogenesis, and enhanced osteogenesis.
Hierarchical tissue regeneration
The hierarchically structured biomaterials are very suitable for hierarchical tissue regeneration, including osteochondral, tendon-to-bone interface, skin, tracheal, and periodontal regeneration.
Osteochondral regeneration. Due to the complexity of hierarchical structures and composition, osteochondral regeneration remains a considerable challenge. The preparation of biomimetic scaffolds with layered structures provided a potential strategy for repairing osteochondria[151]. A study showed that the physicochemical and mechanical properties of the layered PCL scaffold could meet the requirements of the design of bone cartilage scaffolds[151]. ECM hydrogel was incorporated into the upper pore structure, showing excellent cartilage regeneration performance. The lower scaffold possessed a significant osteogenic differentiation due to the continuous release of Mg ions. The repair results in vivo indicated no tissue separation between the regenerated cartilage and subchondral bone. Overall, the integrated double-layer scaffold revealed effective osteochondral repair ability. In addition, Gao et al. prepared a hierarchical scaffold composed of a hydrogel layer on a porous cryogel to simulate the layered structure of osteochondral tissue[152]. The upper layer of hydrogel supplemented high Mg ions and possessed small pores, which could promote cartilage differentiation. At the same time, the bottom frozen gel showed a macroporous and interconnected pore structure with low Mg supplementation, contributing to stem cell migration, matrix mineralization, and osteogenesis. Therefore, this hierarchical double-layer scaffold was expected to achieve optimal regeneration of cartilage and subchondral tissue. Moreover, PCL microsphere-based scaffolds with hierarchical structures were fabricated for osteochondral repair[153]. At the macro level, integrated scaffolds with different channel modes of non-channel, continuous-channel, and inconsecutive-channel had been fabricated. At the micro level, the two layers of the integrated scaffold were composed of small and large PCL microspheres. The experiment results indicated that the inconsecutive-channel scaffold possessed layered structures and gradient interconnected porosity. The cartilage area presented a dense phase that inhibited vascular invasion, while the subchondral bone area produced a porous phase for bone and vascular growth, facilitating the remodeling of osteochondria. Consequently, this work demonstrated that integrated design with hierarchical structures provided a feasible method for repairing cartilage and subchondral bone.
Tendon-to-bone interface regeneration. Tendon-to-bone interface injury is also one of the most common and challenging injuries. The tendon-to-bone interface has a hierarchical structure and composition. Thus, biomaterials with hierarchical structures are widely developed for repairing the tendon-to-bone interface. A biomimetic nanofiber scaffold was successfully constructed to simulate the microstructure of the tendon-bone interface[154]. The curly microstructure mimicked the natural tendon-bone interface ECM, promoting cartilage generation at the interface area. The in vivo results showed that the scaffold induced a continuous translational interface between tendons and bones. Our group created a biomimetic multicellular layered scaffold by designing the spatial distribution of biomimetic ink to simulate the hierarchical structure and cell composition of the tendon-bone interface[155]. It was found that the biomimetic scaffold presented dual functions of inducing tendon differentiation and osteogenic differentiation in vitro and promoted regeneration of the tendon-to-bone interface in vivo. Furthermore, Zhang et al. prepared scaffolds with hierarchical structures, composition, and mechanics to promote tendon-to-bone interface regeneration[156]. The scaffold possessed a three-layer structure that could support the creation of a bone tissue area at the bottom, a transitional tissue area in the middle, and a tendon area at the top. The repair experiment of rotator cuff injury in vivo confirmed that the hierarchically structured scaffold could induce satisfactory graded tendon-bone differentiation properties in the repair interface.
Skin regeneration. The treatment of full-thickness wounds also requires biomaterials with matched structure characteristics. Hierarchical scaffolds can provide a necessary microenvironment for appropriate structural and functional reconstruction of full-thickness skin. Lu et al. prepared layered nanofiber scaffolds with arranged porous structures to promote skin regeneration[157]. It was found that the layered fiber structure promoted cell adhesion and proliferation in the scaffold, while the arranged porous structure facilitated the infiltration of fibroblasts and endothelial cells, thereby promoting angiogenesis and wound healing. In addition, a biodegradable scaffold that mimics the function and structure of three layers of skin was developed for wound healing[158]. The scaffold showed gradient porosity, mechanical properties, and hydrophilicity similar to skin, which could meet the needs of skin regeneration of different levels and was very conducive to wound healing. The scaffold with a highly porous fiber structure and enhanced fiber-to-fiber distance was developed by electrospinning technology[159]. This scaffold could promote cell migration, matrix remodeling, and ECM synthesis. In addition, the utilization of highly porous fiber scaffolds can generate a three-layer skin model including the dermis, epidermis, and subcutaneous tissue, demonstrating the potential of scaffolds with high porosity and moveable fibers as substitutes for constructing tissue models.
Tracheal regeneration. Developing biomimetic tracheal substitutes is a vast challenge in reconstructing segmental tracheal defects[160]. Due to the unique physiological structure of the trachea, there are also certain requirements for the biomaterial structure in tracheal regeneration. In particular, by constructing a hierarchically structured scaffold, the structure of the trachea can be simulated, which is beneficial for tracheal regeneration. Wu et al. successfully developed a human airway-like multilayered tissue based on interpenetrated hierarchical structures[161]. The layered, interconnected porous structure of the scaffold allowed collagen hydrogels to be impregnated into a mutually permeable hybrid network, which could support the culture of epithelial cells. Besides, the scaffold could promote the growth and differentiation of human bronchial epithelium, producing a tracheal tissue analog with the biological structure and function of the human airway. Subsequently, a study prepared a biomimetic trachea using a modular strategy similar in structure and function to natural trachea[162]. Specifically, the porous chondroitin-sulfate-incorporating type-II atelocollagen (COL II/CS) scaffold as a cartilage ring is alternatively stacked on a silicone tube with a porous COL II/CS scaffold to form a biomimetic trachea. In tracheal reconstruction, the biomimetic trachea showed satisfactory therapy effects, demonstrating the effectiveness of scaffolds with layered structures in tracheal regeneration. Moreover, the double-layer tubular scaffold was constructed as an artificial trachea similar to a natural trachea[163]. Chondrocytes derived from iPSC and human bronchial epithelial cells were inoculated into the outer and inner layers of the scaffold, which could enhance the regeneration of the cartilage and mucosal layers, respectively. Correspondingly, the model of artificial tracheal transplantation in vivo showed excellent repair effects.
Periodontal regeneration. It is difficult to reconstruct the complex mineralized/non-mineralized hierarchical structure of periodontal tissue. To this end, a layered double-layer structure was constructed, consisting of intrafibrillarly mineralized collagen scaffolds with porous structures similar to cementum and unmineralized parallel-arranged fibrils simulating periodontal ligaments[111]. The prepared scaffold showed a unique micro/nanostructure, which could simulate the natural periodontal hard/soft tissue interface. The parallel arrangement of the upper structure was able to attract more periodontal ligament stem cells to form fibroblast-like morphology. The biphasic structure successfully induced the differentiation of stem cells into soft and hard periodontal tissue. By recruiting and regulating host stem cells, it contributed to periodontal tissue regeneration [Figure 9]. In addition, hierarchical scaffolds with customized components and structures were developed for the regeneration of the periodontal ligament-bone interface[164]. The incorporation of magnesium phosphate into the upper scaffold improved mechanical properties and facilitated osteogenic differentiation, while the highly arranged PCL fibers in the lower layer could induce ligament regeneration. Two different fiber structures were in the interface area, allowing for coordinated regeneration of multiple tissues. Furthermore, Ma et al. designed a hierarchical biomimetic periodontal patch for periodontal regeneration[165]. Firstly, a columnar hydrogel matrix was prepared to provide specific spatial guidance for cells in its interior and simulate the specific orientation of periodontal ligament fiber bundles. Subsequently, grid scaffolds were added to support the theme structure. Meanwhile, microchannels could allow for nutrient exchange between columnar structures and grids. The bioengineered tissue produced by this patch was very similar to natural periodontal tissue, especially showing different and specific directional fibers in various tooth root areas. Overall, the scaffold indicated hierarchical composition and structure, demonstrating effective simulation of periodontal tissue and excellent regeneration ability.
Figure 9. Hierarchical bilayer structured scaffold for periodontal tissue regeneration. (A) The schematic diagram of manufacturing of hierarchical bilayer architectures. (B) SEM and confocal images of upper and lower layers from the top to the bottom. (C) Parallel-arranged CGF (P-CGF), randomly arranged CGF (R-CGF), and cell arrangement in the intrafibrillarly mineralized collagen (IMC) layer at day 1. (D) Micro-CT, H&E, and Masson trichrome staining of the periodontal tissue regeneration area of different groups. (E) Immunohistochemical staining of CD146, STRO-1, BMP2, COL-1, and VEGFR-1. Arrow: Positive cells. (F) Semiquantitative analysis of positive cell count of (E). Reproduced with permission[111]. Copyright 2021, Publishing services by Elsevier BV on behalf of KeAi Communications Co. Ltd. *P < 0.05.
Other hierarchical tissue regeneration
Hierarchically structured biomaterials have also been developed for other tissue regeneration scenarios, including myocardial regeneration, muscle regeneration, and corneal regeneration. Wang et al. constructed cardiac constructs with highly arranged microstructures and adjustable curvature[166]. The shape of cardiac constructs could be changed as needed to simulate and reconstruct the curved topological structure of myocardial tissue. The optimal microchannel width was determined based on cell morphology and arrangement. Importantly, it was found that uniform distribution of arranged cells and excellent myocardial maturation in curved cardiac constructs. In addition, scaffolds with micro/macro architectures had been developed for myocardial 3D tissue reconstruction[167]. The scaffold was composed of a stackable double-layer structure with linear micro ridge/groove patterns and macro through-hole arrays, which could achieve customizable anisotropy and interconnected free space. It was found that this hierarchical scaffold was able to guide cell arrangement accurately, further achieving 3D pattern reconstruction similar to natural myocardium.
Structures of biomaterials also play an important role in guiding muscle regeneration. For instance, cell arrangement and myotube formation are crucial for muscle tissue regeneration. A hierarchical scaffold composed of microscale and nanoscale morphology structures was prepared to achieve this goal[168]. This hierarchical structure not only enhanced mechanical stability but also induced myotube formation. Meanwhile, highly aligned nanofibers contributed to the alignment of myoblasts, confirming the potential application of scaffolds with hierarchical structures for muscle tissue regeneration. The layered porous structure could provide guiding clues for cell migration and spatial organization, which is conducive to reconstructing the structure and function of targeted tissues. In addition, ECM scaffolds with parallel microchannels were designed to guide muscle regeneration[169]. Skeletal muscle cells could grow longitudinally along microchannels, promoting cell migration and differentiation. The experimental results in vivo demonstrated that porous scaffolds with parallel microchannel structures were able to promote muscle regeneration.
Due to its complex structure and corneal fibroblast transformation, cornea regeneration remains challenging. Grid-shaped microfiber scaffolds with different fiber spacing were prepared, and GelMA hydrogel was injected into the scaffolds[170]. It was found that this structure could simulate stromal structures similar to natural corneal characteristics and induce the regeneration of damaged corneal stroma in vitro and in vivo by maintaining keratinocyte phenotypes and inducing orthogonal arrangement of keratinocytes to secrete specific ECM. Besides, He et al. prepared a bionic epithelial/stroma double dome hydrogel scaffold for corneal regeneration, which consisted of the epithelial layer loaded with rabbit corneal epithelial cells and the orthogonal arrangement of fibrous matrix layers (loaded with rabbit fat-derived MSCs)[171]. The synergistic effect of microstructures and precise positioning of cells provided a satisfactory microenvironment for corneal regeneration, demonstrating the advantages of hierarchically structured scaffolds for corneal regeneration.
CONCLUSION AND PROSPECTIVES
The hierarchical structure of biomaterials is crucial for tissue regeneration, as they can regulate comprehensive cell functions to promote tissue regeneration. In tissue regeneration, the macro and microstructures of biomaterials present the following advantages: (1) The structure can provide appropriate mechanical support, thereby guiding tissue regeneration; (2) The degradation rate can regulated by structures to match with tissue regeneration; (3) Accurately designed structures at different levels can simulate the complex structure of tissues; (4) The structure provides an ideal microenvironment for cell adhesion, diffusion, proliferation, and ECM production; (5) The hierarchical structure enhances the interactions between multiple cells, which is beneficial for multiple cells to participate in tissue repair processes. Given the importance of structures on tissue regeneration, different structured scaffolds have been widely developed for varying tissue regeneration applications. In particular, hierarchically structured scaffolds are suitable for the regeneration of tissues with complex structures, such as osteochondral, tendon-bone, skin, tracheal, and periodontal regeneration. We have summarized representative research works about hierarchically structured scaffolds for different tissue regeneration in Table 2. The performance and advantages of different hierarchical structures were highlighted to demonstrate the function mechanism of hierarchically structured scaffolds in promoting tissue regeneration. Firstly, the mechanical properties, degradability, and other physicochemical properties of the scaffolds can be regulated to provide controllable mechanical support for tissue repair and the optimal growth space of newly formed tissues. In addition, improved bioactivity is crucial for tissue repair. The mechanism of structural regulation on bioactivity is mainly related to the interaction between the structure and cells. Some cells with specific functions, such as endothelial cells, stem cells, and macrophages, can sense changes in topography cues, which, in turn, influence certain cellular functions (targeted differentiation, angiogenesis, and immune regulation) and paracrine effects, which is very beneficial for regulating the repair microenvironment. Overall, tissue repair can proceed well under the multiple signals provided by the structure.
The summary of hierarchically structured scaffolds for tissue regeneration
Hierarchically structured scaffolds | Preparation methods | Performances and advantages | Applications |
Multi-layered scaffolds | 3D bioprinting | Coordinate dynamic mechanical stimulation; mimic native cell composition | Osteochondral tissue[76,151-152]; tendon-bone[155] |
Freeze-drying | Improve mechanical properties; control cell arrangement and differentiation | Periodontium[111] | |
3D printing | Provide structure and function similar to native tissues | Trachea[161-163]; cornea[171] | |
Cast molding | Guide cell arrangement; improve cell metabolism and matrix anabolism | Annulus fibrosus[138] | |
Scaffolds with integrated units | Electrospinning | Mimic the structure of native tissue; improve mechanical and bioactivity | Temporomandibular joint disc[45] |
3D printing | Mimic the structure of native tissue; regulate cell-cell interactions | Bone[144-145,147,150]; flat bone[136] | |
Electrospinning and 3D printing | Mimic the structure of native tissue; guide cell alignment and differentiation | Myocardium[49]; skeletal muscle tissue[122]; adipo tissue[123] | |
Freeze-drying and cast molding | Improve perfusion properties and neurogenesis; direct the growth of neotissues | Peripheral nerve[57,128] | |
Scaffolds with varying length scales | Electrospinning | Mimic the structure of native tissue; improve mechanical strength; enhance cell bioactivity | Bone[48,132]; skin[159] |
3D printing | Mimic the structure of native tissue; enhance cell bioactivity | Osteochondral regeneration[59]; bone[119,127,130] | |
Electrospinning and 3D printing | Mimic the structure of native tissue; guide cell arrangement | Myocardium[168] | |
Freezing drying | Improve mechanical performance and angiogenesis | Soft gingival tissue[58] | |
Femtosecond laser direct writing | Mimic the structure of native tissue; guide cell arrangement | Bone[137] |
Although hierarchically structured biomaterials have been widely studied for tissue regeneration and shown favorable repair effects, several issues still need to be addressed. (1) The preparation of hierarchically structured biomaterials requires appropriate fabrication methods, with 3D printing technology being the most commonly used method. However, the accuracy of 3D printing is limited, especially for some precise surface structures that are difficult to replicate. Therefore, it is crucial to design scaffolds that meet the needs at different scales by combining multiple preparation methods; (2) The structure of biomaterials changes over time. The regulation of these dynamic changes in cell behaviors and tissue regeneration processes may be variable and need further study; (3) In many studies, one of the main purposes of designing hierarchically structured materials is to simulate the structure of complex organizations for guiding tissue regeneration with the envisioned structure. However, the regeneration environment in vivo is relatively complex, and the actual growth of tissues may disrupt the plan. Thus, how to confirm that newborn tissues grow as expected should be considered; (4) 3D bioprinting can print cells into different hierarchical structures. However, the printed cell types are relatively single, insufficient to reflect the biological characteristics of specific tissue structures, and exhibit limited functionality. Organoids refer to 3D multicellular constructs formed by the self-organization of stem cells or organ-specific progenitor cells, which can simulate many structural and functional features of real tissues[172]. Therefore, organoids may serve as biological components when bioprinted with hierarchically structured scaffolds, better promoting tissue regeneration; and (5) The structure of biomaterials can be regulated by some external stimulation, including light, heat, magnetic field, etc. The behavior and function of cells may respond to the dynamic and flexible adjustment of structure. This possesses the potential to develop intelligent biomaterials of bioactivity responding structure for tissue regeneration. To address the limitations of current hierarchically structured biomaterials, several aspects should be considered. Firstly, the design of biomimetic hierarchical structures can be enhanced by combining multiple preparation methods. It will allow for improved resolution of structures, such as pore structures, through the use of advanced preparation techniques. It is important to note that the structure of biomaterials may change over time after implantation. To ensure integrity and compatibility with tissue regeneration, different tissue defects may require specific hierarchical structure retention times. Thus, the composition of biomaterials used to construct hierarchical structures must also be carefully considered, as it can affect scaffold degradation and support structure maintenance. Monitoring specific components of biomaterials can provide valuable insights into the maintenance of scaffold structures in vivo. Furthermore, incorporating organoids as stable and comprehensive cell sources in hierarchical scaffolds offers opportunities for more realistic tissue structure simulation and advantages in promoting tissue regeneration. Exploring whether the developmental influence of organoids in different structures can regulate tissue regeneration is an area of interest for future study. Finally, combining hierarchical structures with external stimuli holds promise in advancing the design of hierarchically structured scaffolds toward a more flexible direction. External stimuli can regulate the structure of scaffolds to align with the tissue regeneration process. This integration of external stimuli and hierarchical structures offers potential for improved scaffold adaptability.
The structures of biomaterials play an essential role in tissue regeneration. The proper structure effectively promotes tissue regeneration through the regulation of different levels of performance. Varying hierarchical structures can improve the performance of the scaffolds by tuning the physicochemical properties and enhancing the bioactivities. Future research can focus on matching the hierarchical structure with the process of tissue regeneration and applying the hierarchical structure to simulate the structural characteristics of natural organizations more realistically and in detail. In addition, the advances in fabrication technologies are key to realizing complex hierarchical structures. It is expected that fabrication technologies and methods can mutually be reinforced to optimize the current types and functions of hierarchical structures. Overall, further efforts are needed to optimize structural design for comprehensive tissue regeneration.
DECLARATIONS
Authors’ contributions
Conceptualization and writing-original draft: Ma W
Writing-review and editing: Yang Z, Lu M, Ma H
Writing-review & editing, supervision, and funding acquisition: Wu C, Lu H
Availability of data and materials
Not applicable.
Financial support and sponsorship
We acknowledge funding support from the National Natural Science Foundation of China (32225028, 32130062), CAS Project for Young Scientists in Basic Research (YSBR073), Shanghai Pilot Program for Basic Research-Chinese Academy of Science, Shanghai Branch (JCYJ-SHFY-2022-003), Shanghai Talent Scholar Program, and the Shanghai Sailing Program (21YF1431300).
Conflicts of interest
All authors declared that there are no conflicts of interest.
Ethical approval and consent to participate
Not applicable.
Consent for publication
Not applicable.
Copyright
© The Author(s) 2024.
REFERENCES
1. Xue J, Qin C, Wu C. 3D printing of cell-delivery scaffolds for tissue regeneration. Regen Biomater 2023;10:rbad032.
2. Bittner SM, Guo JL, Melchiorri A, Mikos AG. Three-dimensional printing of multilayered tissue engineering scaffolds. Mater Today 2018;21:861-74.
3. Li T, Chang J, Zhu Y, Wu C. 3D printing of bioinspired biomaterials for tissue regeneration. Adv Healthc Mater 2020;9:e2000208.
4. Zhou Y, Wu C, Chang J. Bioceramics to regulate stem cells and their microenvironment for tissue regeneration. Mater Today 2019;24:41-56.
5. Gatto ML, Cerqueni G, Groppo R, et al. Improved biomechanical behavior of 316L graded scaffolds for bone tissue regeneration produced by laser powder bed fusion. J Mech Behav Biomed Mater 2023;144:105989.
6. Liu J, Xu C, Xu Y, et al. Bioinspired scaffolds with hierarchical structures for tailored mechanical behaviour and cell migration. Ceram Int 2020;46:24102-9.
7. Zhang H, Huang H, Hao G, et al. 3D Printing Hydrogel Scaffolds with nanohydroxyapatite gradient to effectively repair osteochondral defects in rats. Adv Funct Mater 2021;31:2006697.
8. Apsite I, Stoychev G, Zhang W, Jehnichen D, Xie J, Ionov L. Porous stimuli-responsive self-folding electrospun mats for 4D biofabrication. Biomacromolecules 2017;18:3178-84.
9. Chen Z, Bachhuka A, Han S, et al. Tuning chemistry and topography of nanoengineered surfaces to manipulate immune response for bone regeneration applications. ACS Nano 2017;11:4494-506.
10. Servatan M, Zarrintaj P, Mahmodi G, et al. Zeolites in drug delivery: progress, challenges and opportunities. Drug Discov Today 2020;25:642-56.
11. Carotenuto F, Politi S, Ul Haq A, et al. From soft to hard biomimetic materials: tuning micro/nano-architecture of scaffolds for tissue regeneration. Micromachines 2022;13:780.
12. Xiang Y, Lu L, Kottapalli AGP, Pei Y. Status and perspectives of hierarchical porous carbon materials in terms of high-performance lithium-sulfur batteries. Carbon Energy 2022;4:346-98.
13. Nowicki MA, Castro NJ, Plesniak MW, Zhang LG. 3D printing of novel osteochondral scaffolds with graded microstructure. Nanotechnology 2016;27:414001.
14. Bertsch C, Maréchal H, Gribova V, et al. Biomimetic bilayered scaffolds for tissue engineering: from current design strategies to medical applications. Adv Healthc Mater 2023;12:e2203115.
15. Hayashi K, Kishida R, Tsuchiya A, Ishikawa K. Superiority of triply periodic minimal surface gyroid structure to strut-based grid structure in both strength and bone regeneration. ACS Appl Mater Interfaces 2023;15:34570-7.
16. Wang W, Xiong Y, Zhao R, Li X, Jia W. A novel hierarchical biofunctionalized 3D-printed porous Ti6Al4V scaffold with enhanced osteoporotic osseointegration through osteoimmunomodulation. J Nanobiotechnology 2022;20:68.
17. Lee H, Kim G. Enhanced cellular activities of polycaprolactone/alginate-based cell-laden hierarchical scaffolds for hard tissue engineering applications. J Colloid Interface Sci 2014;430:315-25.
18. Xu Y, Cui W, Zhang Y, et al. Hierarchical micro/nanofibrous bioscaffolds for structural tissue regeneration. Adv Healthc Mater 2017;6:1601457.
19. Norzain NA, Lin W. Fibroblast cell responses to physical cues of the triangular prism micropattern and aligned nanofibrous scaffold for promoting wound closure. Mater Des 2022;220:110864.
20. Liu Y, Yang S, Cao L, Zhang X, Wang J, Liu C. Facilitated vascularization and enhanced bone regeneration by manipulation hierarchical pore structure of scaffolds. Mater Sci Eng C 2020;110:110622.
21. Ribeiro VP, Pina S, Costa JB, et al. Enzymatically cross-linked silk fibroin-based hierarchical scaffolds for osteochondral regeneration. ACS Appl Mater Interfaces 2019;11:3781-99.
22. Hu Y, Wang J, Li X, et al. Facile preparation of bioactive nanoparticle/poly(ε-caprolactone) hierarchical porous scaffolds via 3D printing of high internal phase Pickering emulsions. J Colloid Interface Sci 2019;545:104-15.
23. Xie X, Cai J, Yao Y, et al. A woven scaffold with continuous mineral gradients for tendon-to-bone tissue engineering. Compos B Eng 2021;212:108679.
24. Sun T, Zhu Y, Chen F, et al. Ultralong hydroxyapatite nanowires/collagen scaffolds with hierarchical porous structure, enhanced mechanical properties and excellent cellular attachment. Ceram Int 2017;43:15747-54.
25. Chen Y, Guo C, Manousiouthakis E, et al. Bi-layered tubular microfiber scaffolds as functional templates for engineering human intestinal smooth muscle tissue. Adv Funct Mater 2020;30:2000543.
26. Guzzi EA, Tibbitt MW. Additive manufacturing of precision biomaterials. Adv Mater 2020;32:e1901994.
27. Boydston AJ, Cao B, Nelson A, et al. Additive manufacturing with stimuli-responsive materials. J Mater Chem A 2018;6:20621-45.
28. Zhang L, Wang B, Song B, et al. 3D printed biomimetic metamaterials with graded porosity and tapering topology for improved cell seeding and bone regeneration. Bioact Mater 2023;25:677-88.
29. Liang Z, Pei Y, Chen C, et al. General, vertical, three-dimensional printing of two-dimensional materials with multiscale alignment. ACS Nano 2019;13:12653-61.
30. Feng C, Zhang W, Deng C, et al. 3D printing of lotus root-like biomimetic materials for cell delivery and tissue regeneration. Adv Sci 2017;4:1700401.
31. Yang Z, Xue J, Li T, et al. 3D printing of sponge spicules-inspired flexible bioceramic-based scaffolds. Biofabrication 2022;14:035009.
32. Dong C, Wei H, Zhang X, et al. 3D printed hydrogel/wesselsite-PCL composite scaffold with structural change from core/shell fibers to microchannels for enhanced bone regeneration. Compos B Eng 2022;246:110264.
33. Ligon SC, Liska R, Stampfl J, Gurr M, Mülhaupt R. Polymers for 3D printing and customized additive manufacturing. Chem Rev 2017;117:10212-90.
34. Zhang B, Gui X, Song P, et al. Three-dimensional printing of large-scale, high-resolution bioceramics with micronano inner porosity and customized surface characterization design for bone regeneration. ACS Appl Mater Interfaces 2022;14:8804-15.
35. Zhu H, Li M, Huang X, et al. 3D printed tricalcium phosphate-bioglass scaffold with gyroid structure enhance bone ingrowth in challenging bone defect treatment. Appl Mater Today 2021;25:101166.
36. Zhang H, Zhang H, Xiong Y, Dong L, Li X. Development of hierarchical porous bioceramic scaffolds with controlled micro/nano surface topography for accelerating bone regeneration. Mater Sci Eng C 2021;130:112437.
37. He P, Zhao J, Zhang J, et al. Bioprinting of skin constructs for wound healing. Burns Trauma 2018;6:5.
38. Zhou F, Hong Y, Liang R, et al. Rapid printing of bio-inspired 3D tissue constructs for skin regeneration. Biomaterials 2020;258:120287.
39. Hwangbo H, Lee H, Jin EJ, et al. Bio-printing of aligned GelMa-based cell-laden structure for muscle tissue regeneration. Bioact Mater 2022;8:57-70.
40. Chakraborty J, Fernández-Pérez J, van Kampen KA, et al. Development of a biomimetic arch-like 3D bioprinted construct for cartilage regeneration using gelatin methacryloyl and silk fibroin-gelatin bioinks. Biofabrication 2023;15:035009.
41. Zulkifli MZA, Nordin D, Shaari N, Kamarudin SK. Overview of electrospinning for tissue engineering applications. Polymers 2023;15:2418.
42. Liu Y, Guo Q, Zhang X, Wang Y, Mo X, Wu T. Progress in electrospun fibers for manipulating cell behaviors. Adv Fiber Mater 2023;5:1241-72.
43. Xie X, Chen Y, Wang X, et al. Electrospinning nanofiber scaffolds for soft and hard tissue regeneration. J Mater Sci Technol 2020;59:243-61.
44. He FL, Li DW, He J, et al. A novel layer-structured scaffold with large pore sizes suitable for 3D cell culture prepared by near-field electrospinning. Mater Sci Eng C 2018;86:18-27.
45. Gan Z, Zhao Y, Wu Y, Yang W, Zhao Z, Zhao L. Three-dimensional, biomimetic electrospun scaffolds reinforced with carbon nanotubes for temporomandibular joint disc regeneration. Acta Biomater 2022;147:221-34.
46. Han F, Yu Q, Chu G, et al. Multifunctional nanofibrous scaffolds with angle-ply microstructure and co-delivery capacity promote partial repair and total replacement of intervertebral disc. Adv Healthc Mater 2022;11:e2200895.
47. Pal P, Srivas PK, Dadhich P, Das B, Maulik D, Dhara S. Nano-/Microfibrous cotton-wool-like 3D scaffold with core-shell architecture by emulsion electrospinning for skin tissue regeneration. ACS Biomater Sci Eng 2017;3:3563-75.
48. Wang Z, Wang H, Xiong J, et al. Fabrication and in vitro evaluation of PCL/gelatin hierarchical scaffolds based on melt electrospinning writing and solution electrospinning for bone regeneration. Mater Sci Eng C 2021;128:112287.
49. Liu S, Wang Z, Chen X, et al. Multiscale anisotropic scaffold integrating 3D printing and electrospinning techniques as a heart-on-a-chip platform for evaluating drug-induced cardiotoxicity. Adv Healthc Mater 2023;12:e2300719.
50. Lee HS, Jeon EY, Nam JJ, et al. Development of a regenerative porous PLCL nerve guidance conduit with swellable hydrogel-based microgrooved surface pattern via 3D printing. Acta Biomater 2022;141:219-32.
51. Zheng T, Wu L, Xu J, et al. YR/DFO@DCNT functionalized anisotropic micro/nano composite topography scaffolds for accelerating long-distance peripheral nerve regeneration. Compos B Eng 2022;246:110242.
52. Zheng Y, Wu J, Zhu Y, Wu C. Inorganic-based biomaterials for rapid hemostasis and wound healing. Chem Sci 2022;14:29-53.
53. Wegst UG, Bai H, Saiz E, Tomsia AP, Ritchie RO. Bioinspired structural materials. Nat Mater 2015;14:23-36.
54. Shao G, Hanaor DAH, Shen X, Gurlo A. Freeze casting: from low-dimensional building blocks to aligned porous structures-a review of novel materials, methods, and applications. Adv Mater 2020;32:e1907176.
55. Nelson I, Naleway SE. Intrinsic and extrinsic control of freeze casting. J Mater Res Technol 2019;8:2372-85.
56. Nelson M, Li S, Page SJ, et al. 3D printed silica-gelatin hybrid scaffolds of specific channel sizes promote collagen Type II, Sox9 and aggrecan production from chondrocytes. Mater Sci Eng C 2021;123:111964.
57. Li G, Xue C, Wang H, et al. Spatially featured porous chitosan conduits with micropatterned inner wall and seamless sidewall for bridging peripheral nerve regeneration. Carbohydr Polym 2018;194:225-35.
58. Feng Y, Gao H, Wu D, et al. Biomimetic lamellar chitosan scaffold for soft gingival tissue regeneration. Adv Funct Mater 2021;31:2105348.
59. Deng C, Lin R, Zhang M, et al. Micro/Nanometer-structured scaffolds for regeneration of both cartilage and subchondral bone. Adv Funct Mater 2019;29:1806068.
60. Yin J, Zhong J, Wang J, et al. 3D-printed high-density polyethylene scaffolds with bioactive and antibacterial layer-by-layer modification for auricle reconstruction. Mater Today Bio 2022;16:100361.
61. Song P, Hu C, Pei X, et al. Dual modulation of crystallinity and macro-/microstructures of 3D printed porous titanium implants to enhance stability and osseointegration. J Mater Chem B 2019;7:2865-77.
62. Chai N, Zhang J, Zhang Q, et al. Construction of 3D printed constructs based on microfluidic microgel for bone regeneration. Compos B Eng 2021;223:109100.
63. Xu H, Lv F, Zhang Y, et al. Hierarchically micro-patterned nanofibrous scaffolds with a nanosized bio-glass surface for accelerating wound healing. Nanoscale 2015;7:18446-52.
64. Li T, Zhai D, Ma B, et al. 3D printing of hot dog-like biomaterials with hierarchical architecture and distinct bioactivity. Adv Sci 2019;6:1901146.
65. Wu S, Liu X, Yeung KW, Liu C, Yang X. Biomimetic porous scaffolds for bone tissue engineering. Mater Sci Eng R Rep 2014;80:1-36.
66. Li Y, Jahr H, Pavanram P, et al. Additively manufactured functionally graded biodegradable porous iron. Acta Biomater 2019;96:646-61.
67. Yu X, Hu Y, Zou L, et al. A bilayered scaffold with segregated hydrophilicity-hydrophobicity enables reconstruction of goat hierarchical temporomandibular joint condyle cartilage. Acta Biomater 2021;121:288-302.
68. Yang Y, Li X, Chu M, et al. Electrically assisted 3D printing of nacre-inspired structures with self-sensing capability. Sci Adv 2019;5:eaau9490.
69. Tabard L, Garnier V, Prud’homme E, et al. Robocasting of highly porous ceramics scaffolds with hierarchized porosity. Addit Manuf 2021;38:101776.
70. Zhang M, Lin R, Wang X, et al. 3D printing of Haversian bone-mimicking scaffolds for multicellular delivery in bone regeneration. Sci Adv 2020;6:eaaz6725.
71. Jia G, Huang H, Niu J, et al. Exploring the interconnectivity of biomimetic hierarchical porous Mg scaffolds for bone tissue engineering: effects of pore size distribution on mechanical properties, degradation behavior and cell migration ability. J Magnes Alloy 2021;9:1954-66.
72. Zhang Q, Ma L, Ji X, et al. High-strength hydroxyapatite scaffolds with minimal surface macrostructures for load-bearing bone regeneration. Adv Funct Mater 2022;32:2204182.
73. Meng C, Tang D, Liu X, et al. Heterogeneous porous PLLA/PCL fibrous scaffold for bone tissue regeneration. Int J Biol Macromol 2023;235:123781.
74. Nitti P, Palazzo B, Gallo N, Scalera F, Sannino A, Gervaso F. Smooth-rough asymmetric PLGA structure made of dip coating membrane and electrospun nanofibrous scaffolds meant to be used for guided tissue regeneration of periodontium. Polym Eng Sci 2022;62:2061-9.
75. Fan Z, Liu H, Ding Z, Xiao L, Lu Q, Kaplan DL. Simulation of cortical and cancellous bone to accelerate tissue regeneration. Adv Funct Mater 2023;33:2301839.
76. Li T, Ma Z, Zhang Y, et al. Regeneration of humeral head using a 3D bioprinted anisotropic scaffold with dual modulation of endochondral ossification. Adv Sci 2023;10:e2205059.
77. Wang Y, Liu F, Wang N, et al. Bioinspired stretchable helical nanofiber yarn scaffold for locomotive tissue dynamic regeneration. Matter 2022;5:4480-501.
78. No YJ, Castilho M, Ramaswamy Y, Zreiqat H. Role of biomaterials and controlled architecture on tendon/ligament repair and regeneration. Adv Mater 2020;32:e1904511.
79. Xie X, Xu J, Lin J, et al. A regeneration process-matching scaffold with appropriate dynamic mechanical properties and spatial adaptability for ligament reconstruction. Bioact Mater 2022;13:82-95.
80. Arun Y, Ghosh R, Domb AJ. Biodegradable hydrophobic injectable polymers for drug delivery and regenerative medicine. Adv Funct Mater 2021;31:2010284.
81. Ma C, Jing Y, Sun H, Liu X. Hierarchical nanofibrous microspheres with controlled growth factor delivery for bone regeneration. Adv Healthc Mater 2015;4:2699-708.
82. Xu C, Wu F, Yang J, et al. 3D printed long-term structurally stable bioceramic dome scaffolds with controllable biodegradation favorable for guided bone regeneration. Chem Eng J 2022;450:138003.
83. Cai P, Lu S, Yu J, et al. Injectable nanofiber-reinforced bone cement with controlled biodegradability for minimally-invasive bone regeneration. Bioact Mater 2023;21:267-83.
84. Sun Y, You Y, Jiang W, Wang B, Wu Q, Dai K. 3D bioprinting dual-factor releasing and gradient-structured constructs ready to implant for anisotropic cartilage regeneration. Sci Adv 2020;6:eaay1422.
85. Kim JA, Lim J, Naren R, Yun HS, Park EK. Effect of the biodegradation rate controlled by pore structures in magnesium phosphate ceramic scaffolds on bone tissue regeneration in vivo. Acta Biomater 2016;44:155-67.
86. Shi X, Nommeots-nomm A, Todd NM, et al. Bioactive glass scaffold architectures regulate patterning of bone regeneration in vivo. Appl Mater Today 2020;20:100770.
87. Li Y, Li J, Jiang S, et al. The design of strut/TPMS-based pore geometries in bioceramic scaffolds guiding osteogenesis and angiogenesis in bone regeneration. Mater Today Bio 2023;20:100667.
88. Cao G, He W, Fan Y, Li X. Exploring the match between the degradation of the ECM-based composites and tissue remodeling in a full-thickness abdominal wall defect model. Biomater Sci 2021;9:7895-910.
89. Vatankhah E, Prabhakaran MP, Ramakrishna S. Biomimetic microenvironment complexity to redress the balance between biodegradation and de novo matrix synthesis during early phase of vascular tissue engineering. Mater Sci Eng C 2017;81:39-47.
90. Dou Y, Huang J, Xia X, et al. A hierarchical scaffold with a highly pore-interconnective 3D printed PLGA/n-HA framework and an extracellular matrix like gelatin network filler for bone regeneration. J Mater Chem B 2021;9:4488-501.
91. Wang C, Lai J, Li K, et al. Cryogenic 3D printing of dual-delivery scaffolds for improved bone regeneration with enhanced vascularization. Bioact Mater 2021;6:137-45.
92. Zhou X, Qian Y, Chen L, et al. Flowerbed-inspired biomimetic scaffold with rapid internal tissue infiltration and vascularization capacity for bone repair. ACS Nano 2023;17:5140-56.
93. Wang Y, Ling C, Chen J, et al. 3D-printed composite scaffold with gradient structure and programmed biomolecule delivery to guide stem cell behavior for osteochondral regeneration. Biomater Adv 2022;140:213067.
94. Wu L, Li J, Wang Y, et al. Engineered hierarchical microdevices enable pre-programmed controlled release for postsurgical and unresectable cancer treatment. Adv Mater 2023;35:e2305529.
95. Xue J, Ma H, Song E, et al. Bamboo-based biomaterials for cell transportation and bone integration. Adv Healthc Mater 2022;11:e2200287.
96. Shen Y, Wang X, Wang Y, et al. Bilayer silk fibroin/sodium alginate scaffold promotes vascularization and advances inflammation stage in full-thickness wound. Biofabrication 2022;14:035016.
97. Patel A, Mukundan S, Wang W, et al. Carbon-based hierarchical scaffolds for myoblast differentiation: synergy between nano-functionalization and alignment. Acta Biomater 2016;32:77-88.
98. Ul Haq A, Carotenuto F, De Matteis F, et al. Intrinsically conductive polymers for striated cardiac muscle repair. Int J Mol Sci 2021;22:8550.
99. Diedkova K, Pogrebnjak AD, Kyrylenko S, et al. Polycaprolactone-MXene nanofibrous scaffolds for tissue engineering. ACS Appl Mater Interfaces 2023;15:14033-47.
100. Ermis M, Antmen E, Hasirci V. Micro and nanofabrication methods to control cell-substrate interactions and cell behavior: a review from the tissue engineering perspective. Bioact Mater 2018;3:355-69.
101. Werner M, Blanquer SB, Haimi SP, et al. Surface curvature differentially regulates stem cell migration and differentiation via altered attachment morphology and nuclear deformation. Adv Sci 2017;4:1600347.
102. Wu L, Zhou C, Zhang B, et al. Construction of biomimetic natural wood hierarchical porous-structure bioceramic with micro/nanowhisker coating to modulate cellular behavior and osteoinductive activity. ACS Appl Mater Interfaces 2020;12:48395-407.
103. Zarrintaj P, Mahmodi G, Manouchehri S, et al. Zeolite in tissue engineering: opportunities and challenges. MedComm 2020;1:5-34.
104. Zhang R, Han S, Ren N, et al. Topographical regulation of stem cell differentiation by plant-derived micro/nanostructures. Nanoscale 2020;12:18305-12.
105. Xia D, Wang Y, Wu R, et al. The effect of pore size on cell behavior in mesoporous bioglass scaffolds for bone regeneration. Appl Mater Today 2022;29:101607.
106. Xie W, Ouyang R, Wang H, Zhou C. Construction and biocompatibility of three-dimensional composite polyurethane scaffolds in liquid crystal state. ACS Biomater Sci Eng 2020;6:2312-22.
107. Hou Y, Xie W, Yu L, et al. Surface roughness gradients reveal topography-specific mechanosensitive responses in human mesenchymal stem cells. Small 2020;16:e1905422.
108. Lu K, Qian Y, Gong J, et al. Biofabrication of aligned structures that guide cell orientation and applications in tissue engineering. Bio-des Manuf 2021;4:258-77.
109. Yeo M, Kim GH. Anisotropically aligned cell-laden nanofibrous bundle fabricated via cell electrospinning to regenerate skeletal muscle tissue. Small 2018;14:e1803491.
110. Razali NA, Lin WC, Norzain NA, Yu ZW. Controlling cell elongation and orientation by using microstructural nanofibre scaffolds for accelerating tissue regeneration. Mater Sci Eng C 2021;128:112321.
111. Yu M, Luo D, Qiao J, et al. A hierarchical bilayer architecture for complex tissue regeneration. Bioact Mater 2022;10:93-106.
112. Okeyo KO, Kibe Y, Adachi T. Controlling macroscale cell alignment in self-organized cell sheets by tuning the microstructure of adhesion-limiting micromesh scaffolds. Mater Today Adv 2021;12:100194.
113. Feng B, Zhang M, Qin C, et al. 3D printing of conch-like scaffolds for guiding cell migration and directional bone growth. Bioact Mater 2023;22:127-40.
114. Yang D, Zhao Z, Bai F, Wang S, Tomsia AP, Bai H. Promoting cell migration in tissue engineering scaffolds with graded channels. Adv Healthc Mater 2017:6.
115. Bai H, Wang D, Delattre B, et al. Biomimetic gradient scaffold from ice-templating for self-seeding of cells with capillary effect. Acta Biomater 2015;20:113-9.
116. Feng X, Xu P, Shen T, Zhang Y, Ye J, Gao C. Influence of pore architectures of silk fibroin/collagen composite scaffolds on the regeneration of osteochondral defects in vivo. J Mater Chem B 2020;8:391-405.
117. Wang K, Man K, Liu J, Meckes B, Yang Y. Dissecting physical and biochemical effects in nanotopographical regulation of cell behavior. ACS Nano 2023;17:2124-33.
118. Yang C, Wang X, Ma B, et al. 3D-printed bioactive Ca3SiO5 bone cement scaffolds with nano surface structure for bone regeneration. ACS Appl Mater Interfaces 2017;9:5757-67.
119. Li M, Ma H, Han F, et al. Microbially catalyzed biomaterials for bone regeneration. Adv Mater 2021;33:e2104829.
120. Long EG, Buluk M, Gallagher MB, Schneider JM, Brown JL. Human mesenchymal stem cell morphology, migration, and differentiation on micro and nano-textured titanium. Bioact Mater 2019;4:249-55.
121. Xue P, Liu W, Gu Z, et al. Graded protein/PEG nanopattern arrays: well-defined gradient biomaterials to induce basic cellular behaviors. ACS Appl Mater Interfaces 2019;11:1595-603.
122. Yeo M, Lee H, Kim GH. Combining a micro/nano-hierarchical scaffold with cell-printing of myoblasts induces cell alignment and differentiation favorable to skeletal muscle tissue regeneration. Biofabrication 2016;8:035021.
123. Meng Z, Mu X, He J, Zhang J, Ling R, Li D. Embedding aligned nanofibrous architectures within 3D-printed polycaprolactone scaffolds for directed cellular infiltration and tissue regeneration. Int J Extrem Manuf 2023;5:025001.
124. Kong B, Sun L, Liu R, et al. Recombinant human collagen hydrogels with hierarchically ordered microstructures for corneal stroma regeneration. Chem Eng J 2022;428:131012.
125. Jiang S, Wang M, Wang Z, et al. Radially porous nanocomposite scaffolds with enhanced capability for guiding bone regeneration in vivo. Adv Funct Mater 2022;32:2110931.
126. Eichholz KF, Freeman FE, Pitacco P, et al. Scaffold microarchitecture regulates angiogenesis and the regeneration of large bone defects. Biofabrication 2022;14:045013.
127. Ha Y, Ma X, Li S, et al. Bone microenvironment-mimetic scaffolds with hierarchical microstructure for enhanced vascularization and bone regeneration. Adv Funct Mater 2022;32:2200011.
128. Lu Q, Zhang F, Cheng W, et al. Nerve guidance conduits with hierarchical anisotropic architecture for peripheral nerve regeneration. Adv Healthc Mater 2021;10:e2100427.
129. Li G, Zheng T, Wu L, et al. Bionic microenvironment-inspired synergistic effect of anisotropic micro-nanocomposite topology and biology cues on peripheral nerve regeneration. Sci Adv 2021;7:eabi5812.
130. Yu X, Wang Y, Zhang M, et al. 3D printing of gear-inspired biomaterials: immunomodulation and bone regeneration. Acta Biomater 2023;156:222-33.
131. Zhang Z, Xie Y, Pan H, Huang L, Zheng X. Influence of patterned titanium coatings on polarization of macrophage and osteogenic differentiation of bone marrow stem cells. J Biomater Appl 2018;32:977-86.
132. He Y, Tian M, Li X, et al. A hierarchical-structured mineralized nanofiber scaffold with osteoimmunomodulatory and osteoinductive functions for enhanced alveolar bone regeneration. Adv Healthc Mater 2022;11:e2102236.
133. Liu Y, Cao L, Zhang S, Ji L, Wang J, Liu C. Effect of hierarchical porous scaffold on osteoimmunomodulation and bone formation. Appl Mater Today 2020;20:100779.
134. Yin Y, He X, Wang J, et al. Pore size-mediated macrophage M1-to-M2 transition influences new vessel formation within the compartment of a scaffold. Appl Mater Today 2020;18:100466.
135. Zheng X, Chen L, Tan J, et al. Effect of micro/nano-sheet array structures on the osteo-immunomodulation of macrophages. Regen Biomater 2022;9:rbac075.
136. Mao R, Lai Y, Li D, et al. Flow channel performance in 3D printed hydroxyapatite scaffolds to improve metabolism and tissue ingrowth in flat bone repair. Compos B Eng 2023;259:110727.
137. Gu H, Zhu Y, Yang J, et al. Liver-inspired polyetherketoneketone scaffolds simulate regenerative signals and mobilize anti-inflammatory reserves to reprogram macrophage metabolism for boosted osteoporotic osseointegration. Adv Sci 2023;10:e2302136.
138. Zhang W, Wang H, Chu G, et al. Mechanically conditioned multilayered angle-ply collagen scaffolds promote annulus fibrosus regeneration. Appl Materi Today 2023;31:101751.
139. He D, Li H. Biomaterials affect cell-cell interactions in vitro in tissue engineering. J Mater Sci Technol 2021;63:62-72.
140. Ren X, Gao X, Cheng Y, et al. Maintenance of multipotency of bone marrow mesenchymal stem cells on poly(ε-caprolactone) nanoneedle arrays through the enhancement of cell-cell interaction. Front Bioeng Biotechnol 2022;10:1076345.
141. Lutzweiler G, Barthes J, Koenig G, et al. Modulation of cellular colonization of porous polyurethane scaffolds via the control of pore interconnection size and nanoscale surface modifications. ACS Appl Mater Interfaces 2019;11:19819-29.
142. Coyle S, Doss B, Huo Y, et al. Cell alignment modulated by surface nano-topography - roles of cell-matrix and cell-cell interactions. Acta Biomater 2022;142:149-59.
143. Gautam V, Naureen S, Shahid N, et al. Engineering highly interconnected neuronal networks on nanowire scaffolds. Nano Lett 2017;17:3369-75.
144. Zhang M, Qin C, Wang Y, et al. 3D printing of tree-like scaffolds for innervated bone regeneration. Addit Manuf 2022;54:102721.
145. Wang Y, Wang Z, Yu X, et al. 3D-Printing of succulent plant-like scaffolds with beneficial cell microenvironments for bone regeneration. J Mater Chem B 2023;11:5523-36.
146. Zhang B, Zhang M, Sun Y, Li M, Han F, Wu C. Haversian bone-mimicking bioceramic scaffolds enhancing MSC-macrophage osteo-imunomodulation. Prog Nat Sci Mater Int 2021;31:883-90.
147. Zhang B, Han F, Wang Y, et al. Cells-micropatterning biomaterials for immune activation and bone regeneration. Adv Sci 2022;9:e2200670.
148. Li L, Wang P, Liang H, et al. Design of a Haversian system-like gradient porous scaffold based on triply periodic minimal surfaces for promoting bone regeneration. J Adv Res 2023;54:89-104.
149. Shen M, Li Y, Lu F, et al. Bioceramic scaffolds with triply periodic minimal surface architectures guide early-stage bone regeneration. Bioact Mater 2023;25:374-86.
150. Zhang J, Tong D, Song H, et al. Osteoimmunity-regulating biomimetically hierarchical scaffold for augmented bone regeneration. Adv Mater 2022;34:e2202044.
151. Li C, Zhang W, Nie Y, et al. Integrated and bifunctional bilayer 3D printing scaffold for osteochondral defect repair. Adv Funct Mater 2023;33:2214158.
152. Gao C, Dai W, Wang X, et al. Magnesium gradient-based hierarchical scaffold for dual-lineage regeneration of osteochondral defect. Adv Funct Materials 2023;33:2304829.
153. Gu X, Zha Y, Li Y, et al. Integrated polycaprolactone microsphere-based scaffolds with biomimetic hierarchy and tunable vascularization for osteochondral repair. Acta Biomater 2022;141:190-7.
154. Wang L, Zhu T, Kang Y, et al. Crimped nanofiber scaffold mimicking tendon-to-bone interface for fatty-infiltrated massive rotator cuff repair. Bioact Mater 2022;16:149-61.
155. Du L, Qin C, Zhang H, et al. Multicellular bioprinting of biomimetic inks for tendon-to-bone regeneration. Adv Sci 2023;10:e2301309.
156. Zhang X, Song W, Han K, et al. Three-dimensional bioprinting of a structure-, composition-, and mechanics-graded biomimetic scaffold coated with specific decellularized extracellular matrix to improve the tendon-to-bone healing. ACS Appl Mater Interfaces 2023;15:28964-80.
157. Lu G, Ding Z, Wei Y, Lu X, Lu Q, Kaplan DL. Anisotropic Biomimetic Silk Scaffolds for Improved Cell Migration and Healing of Skin Wounds. ACS Appl Mater Interfaces 2018;10:44314-23.
158. Ghosh S, Haldar S, Gupta S, et al. Single unit functionally graded bioresorbable electrospun scaffold for scar-free full-thickness skin wound healing. Biomater Adv 2022;139:212980.
159. Weigel T, Malkmus C, Weigel V, et al. Fully synthetic 3D fibrous scaffolds for stromal tissues-replacement of animal-derived scaffold materials demonstrated by multilayered skin. Adv Mater 2022;34:e2106780.
160. Gao E, Li G, Cao R, et al. Bionic tracheal tissue regeneration using a ring-shaped scaffold comprised of decellularized cartilaginous matrix and silk fibroin. Compos B Eng 2022;229:109470.
161. Wu L, Magaz A, Huo S, et al. Human airway-like multilayered tissue on 3D-TIPS printed thermoresponsive elastomer/collagen hybrid scaffolds. Acta Biomater 2020;113:177-95.
162. Xu Y, Dai J, Zhu X, et al. Biomimetic Trachea Engineering via a Modular Ring Strategy Based on Bone-Marrow Stem Cells and Atelocollagen for Use in Extensive Tracheal Reconstruction. Adv Mater 2022;34:e2106755.
163. Kim IG, Park SA, Lee SH, et al. Transplantation of a 3D-printed tracheal graft combined with iPS cell-derived MSCs and chondrocytes. Sci Rep 2020;10:4326.
164. Golafshan N, Castilho M, Daghrery A, et al. Composite graded melt electrowritten scaffolds for regeneration of the periodontal ligament-to-bone interface. ACS Appl Mater Interfaces 2023;15:12735-49.
165. Ma Y, Yang X, Chen Y, et al. Biomimetic peridontium patches for functional periodontal regeneration. Adv Healthc Mater 2023;12:e2202169.
166. Wang Y, Cui H, Wang Y, et al. 4D printed cardiac construct with aligned myofibers and adjustable curvature for myocardial regeneration. ACS Appl Mater Interfaces 2021;13:12746-58.
167. Zhang W, Wang Z, Xie C, et al. Scaffold with micro/macro-architecture for myocardial alignment engineering into complex 3D cell patterns. Adv Healthc Mater 2019;8:e1901015.
168. Yeo M, Kim G. Nano/microscale topographically designed alginate/PCL scaffolds for inducing myoblast alignment and myogenic differentiation. Carbohydr Polym 2019;223:115041.
169. Zhu M, Li W, Dong X, et al. In vivo engineered extracellular matrix scaffolds with instructive niches for oriented tissue regeneration. Nat Commun 2019;10:4620.
170. Kong B, Chen Y, Liu R, et al. Fiber reinforced GelMA hydrogel to induce the regeneration of corneal stroma. Nat Commun 2020;11:1435.
171. He B, Wang J, Xie M, et al. 3D printed biomimetic epithelium/stroma bilayer hydrogel implant for corneal regeneration. Bioact Mater 2022;17:234-47.
173. Hu X, Xu J, Li W, et al. Therapeutic “tool” in reconstruction and regeneration of tissue engineering for osteochondral repair. Appl Biochem Biotechnol 2020;191:785-809.
174. Lei T, Zhang T, Ju W, et al. Biomimetic strategies for tendon/ligament-to-bone interface regeneration. Bioact Mater 2021;6:2491-510.
175. Yu JR, Navarro J, Coburn JC, et al. Current and future perspectives on skin tissue engineering: key features of biomedical research, translational assessment, and clinical application. Adv Healthc Mater 2019;8:e1801471.
176. Dhasmana A, Singh A, Rawal S. Biomedical grafts for tracheal tissue repairing and regeneration “tracheal tissue engineering: an overview”. J Tissue Eng Regen Med 2020;14:653-72.
177. Woo HN, Cho YJ, Tarafder S, Lee CH. The recent advances in scaffolds for integrated periodontal regeneration. Bioact Mater 2021;6:3328-42.
Cite This Article
Export citation file: BibTeX | RIS
OAE Style
Ma W, Yang Z, Lu M, Ma H, Wu C, Lu H. Hierarchically structured biomaterials for tissue regeneration. Microstructures 2024;4:2024014. http://dx.doi.org/10.20517/microstructures.2023.61
AMA Style
Ma W, Yang Z, Lu M, Ma H, Wu C, Lu H. Hierarchically structured biomaterials for tissue regeneration. Microstructures. 2024; 4(2): 2024014. http://dx.doi.org/10.20517/microstructures.2023.61
Chicago/Turabian Style
Ma, Wenping, Zhibo Yang, Mingxia Lu, Hongshi Ma, Chengtie Wu, Hongxu Lu. 2024. "Hierarchically structured biomaterials for tissue regeneration" Microstructures. 4, no.2: 2024014. http://dx.doi.org/10.20517/microstructures.2023.61
ACS Style
Ma, W.; Yang Z.; Lu M.; Ma H.; Wu C.; Lu H. Hierarchically structured biomaterials for tissue regeneration. Microstructures. 2024, 4, 2024014. http://dx.doi.org/10.20517/microstructures.2023.61
About This Article
Special Issue
Copyright
Data & Comments
Data
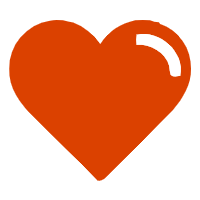

Comments
Comments must be written in English. Spam, offensive content, impersonation, and private information will not be permitted. If any comment is reported and identified as inappropriate content by OAE staff, the comment will be removed without notice. If you have any queries or need any help, please contact us at support@oaepublish.com.